Since the measurement of intrapulmonary shunt requires ventilation with pure oxygen, we usually perform this global daily assessment, setting the ventilator and sweep gas FiO2 to 100 %. On the contrary, during the day, we prefer to assess arterial gases at the clinical FiO2 and to continuously monitor the SvO2.
All these parameters are critical to understand and monitor the contribution of patient lungs to oxygenation and CO2 removal: we will discuss these two functions separately, since they have different underlying physiological mechanisms, as suggested by Kolobow and Gattinoni in the late 1970s [1, 2].
21.2 Oxygenation
Hypoxemia, deficiency of oxygen in arterial blood, is a hallmark of ARDS; quantification of the degree of hypoxemia is critical to assess the severity of the disease and prevent tissue hypoxia. It is difficult to define hypoxemia based on a single PaO2 threshold value. However, when PaO2 is above 60 mmHg, the hemoglobin dissociation curve is almost flat, arterial hemoglobin oxygen saturation (aO2Hb) is higher than 90 %, and the oxygen content of arterial blood is close to the maximum for a given hemoglobin content. Otherwise, PaO2 levels below 40 mmHg, corresponding to an aO2Hb lower than 75 %, invariably result in tissue hypoxia [3]. Hence, several authors [4, 5] suggest a target PaO2 value between 50 and 60 mmHg or a target aO2Hb between 85 and 95 %.
Since in ARDS patients severe hypoxemia is mainly caused by intrapulmonary shunt (see below), VV ECMO, increasing mixed venous oxygen content, may efficiently improve arterial oxygenation if the extracorporeal blood flow is adequate. If the native lung function is completely compromised (i.e., if the intrapulmonary shunt fraction approaches 100 %), an extracorporeal blood flow higher than 4–4.5 l/min (in the absence of extracorporeal blood flow recirculation) will be required to support oxygenation: in such scenario, the arterial oxygen content would be close to the mixed venous one, since NL does not contribute to gas exchange, and therefore oxygenation is completely dependent on ML function (see Fig. 21.1). When the native lung function improves, the difference between arterial and mixed venous oxygen content increases, which further stresses the importance of continuously monitoring mixed venous blood.
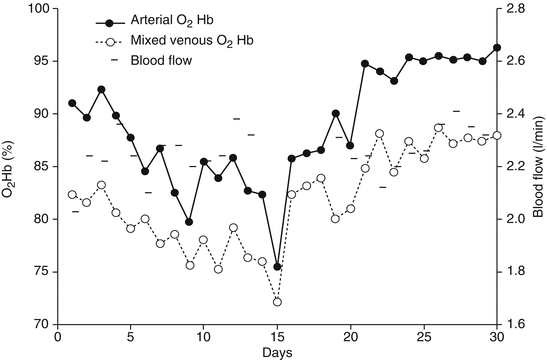
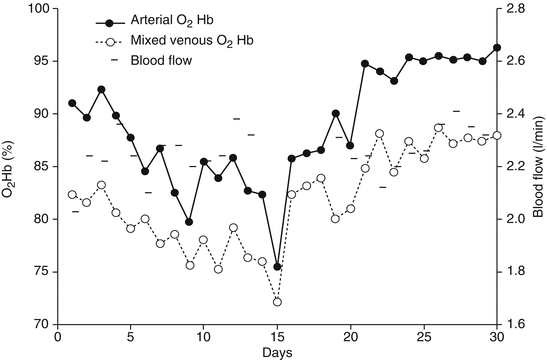
Fig. 21.1
Arterial and mixed venous O2Hb saturation during days in a patient successfully treated with VV ECMO. After approximately 18 days, the patient improved, and, despite a reduction in extracorporeal blood flow, both arterial and mixed venous O2Hb saturation ameliorated
21.2.1 PaO2 and PaO2/FiO2
A variety of indices have been introduced to evaluate oxygenation: the PaO2/FiO2 ratio, the difference between alveolar and arterial PO2, the intrapulmonary shunt fraction, and the oxygenation index. Since the definition of ARDS proposed in 1994 by the American-European Consensus Conference (AECC) [6], PaO2/FiO2 ratio is the more commonly employed index and has been used to differentiate patients with acute lung injury (ALI) (PaO2/FiO2 <300 mmHg) from those with acute respiratory distress syndrome (ARDS) (PaO2/FiO2 <200 mmHg). The PaO2/FiO2 ratio also appears in the new ARDS definition (proposed in 2011 as the “Berlin” definition), where it is used to define three categories of respiratory insufficiency: mild (200 mmHg <PaO2/FiO2 ≤300 mmHg), moderate (100 mmHg <PaO2/FiO2 ≤200 mmHg), and severe (PaO2/FiO2 ≤100 mmHg) [7]. Such degrees of severity were associated with different mortality and duration of mechanical ventilation, although the prognostic role of PaO2/FiO2 ratio in ARDS patients remains to be investigated since previous publications did not show any association between severity of hypoxemia and patient outcome [8, 9]. Such conflicting results can be explained by the fact that the PaO2/FiO2 ratio is strongly influenced by the FiO2 at which it is measured. In patients with moderate (<30 %) intrapulmonary shunt, the PaO2 is greatly affected by FiO2 and their relationship appears to be nonlinear: in particular, the PaO2/FiO2 ratio is greater at both the extremities of the FiO2 range compared to intermediate FiO2 values [10, 11]. Important variations of FiO2 may therefore significantly alter the PaO2/FiO2 ratio, leading to different classifications of the disease [12]. On the other hand, in patients with intrapulmonary shunt higher than 30 %, who may require VV ECMO support, PaO2 is relatively independent from FiO2 and may be a good indicator of lung function; however, also the PaO2/FiO2 ratio appears almost constant, since the commonly employed FiO2 is generally elevated. The average PaO2/FiO2 ratio at enrolment in the “CESAR” ECMO trial was 76 mmHg [13], just slightly higher than that reported by the Italian ECMO network (ECMOnet) in 153 critically ill patients before ECMO institution (63 mmHg) [14].
When the patient has a considerable intrapulmonary shunt and remains hypoxemic even during VV ECMO, PaO2 itself is a good indicator of the native lung condition. Figure 21.2 depicts the variation of PaO2 in two patients undergoing VV ECMO, recorded during the daily assessment with FiO2 100 %. In one patient (solid circles), PaO2 remained always below 50 mmHg despite the maximization of the extracorporeal support (blood flow ranged between 3 and 3.5 l/min), because the intrapulmonary shunt was almost 100 %; the second patient (open circles) showed a significant PaO2 improvement which allowed disconnection from the bypass.
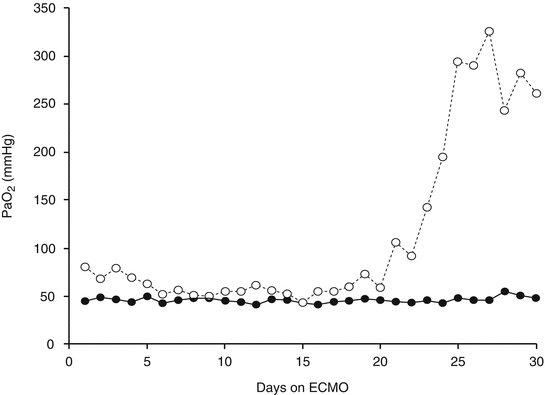
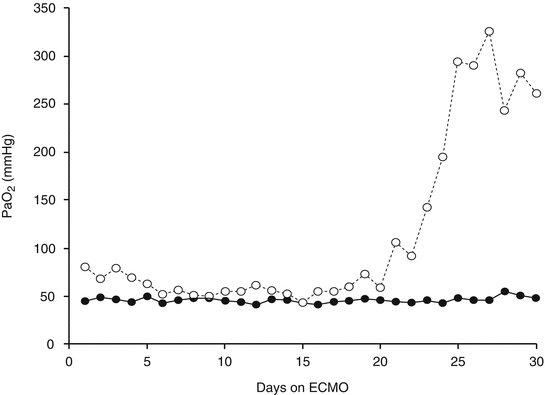
Fig. 21.2
PaO2 over time of two patients on VV ECMO for severe respiratory failure, FiO2 100 %. Open circles: the patient was successfully disconnected from the ECMO. Solid circles: the patient did not survive, his intrapulmonary shunt was always above 95 %, and the PaO2 was always lower than 50 mmHg in spite of an extracorporeal BF always higher than 3 l/min
21.2.2 Oxygenation Index
Although PaO2 and possibly also the PaO2/FiO2 seem good indicators of oxygenation, they do not take into account the cost in terms of positive pressures that must be applied by the ventilator. The oxygenation index (OI) overcomes this limitation, including the mean airway pressure (PAW) value in its calculation as shown in Table. 21.1 [15, 16]. The OI has gained widespread popularity in neonatal and pediatric ECMO patients, but it has recently been proposed also in adults: values above 30 are usually considered an indication for ECMO institution. For example, among the 60 critically ill patients admitted to the Italian ECMOnet ICUs and treated with ECMO support, the median OI (cmH2O/mmHg) was 36.3 and 33.9, respectively, for patients with or without influenza A H1N1 [14].
21.2.3 Membrane Lung and Natural Lung Oxygen Supply
PaO2, PaO2/FiO2 ratio, and the OI do not take into account the oxygen supplied by the ECMO support: indeed, during VV ECMO arterial oxygenation depends on the oxygen added to the blood by the ML (V’O2ML) and by the NL (V’O2NL). At equilibrium, the sum of V’O2ML and V’O2NL equals the total body oxygen consumption. Figure 21.3 shows an example of trends of V’O2ML and V’O2NL in two patients. Panel a: an increase in V’O2NL was observed after 8 days and eventually allowed to reduce the extracorporeal support and successfully disconnect the patient from the ECMO. In panel b, V’O2NL showed a constant decline, and eventually after 15 days of ECMO, the lungs were actually extracting oxygen from the blood resulting in a negative V’O2NL.
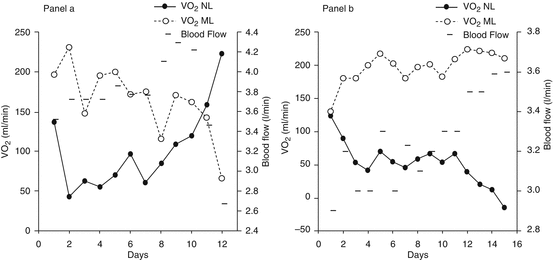
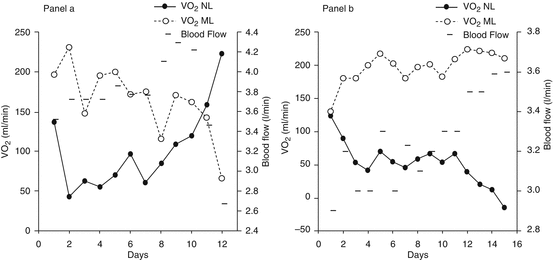
Fig. 21.3
Panel (a): V’O2ML and V’O2NL in a patient disconnected from the VV ECMO after 12 days. Panel (b): V’O2ML and V’O2NL in a patient who died during VV ECMO. Measuring the relative contribution of the NL and the ML allows to monitor the evolution of the native lung disease over time. In panel (b), V’O2NL eventually became negative, since NL shunt was 100 % and the lungs were extracting oxygen from the blood
21.2.4 Intrapulmonary Shunt
Intrapulmonary shunt has been preferred by some authors [11, 17–19] to describe oxygenation in ARDS patients, even if its calculation requires the placement of a pulmonary arterial catheter for mixed venous blood sampling. Intrapulmonary shunt is described as the fraction of cardiac output perfusing nonventilated alveoli and therefore not participating to gas exchange. In healthy subjects, only a small fraction of the cardiac output is shunted, due to perfusion of bronchial tissue and a small amount of coronary venous blood. An intrapulmonary shunt fraction higher than 10 % of the cardiac output results in hypoxemia, since the non-shunted blood, due to the peculiar form of the oxyhemoglobin dissociation curve, is not able to load the extra amount of oxygen required to fully saturate the shunted blood. The nomenclature is not strictly defined, but usually the term “true shunt” is referred to the areas with zero ventilation/perfusion ratio and is calculated as showed in Table. 21.1 while breathing pure oxygen. “Venous admixture” is obtained from the same equation while breathing an oxygen concentration lower than 100 % and embeds three components: ventilation/perfusion mismatch, diffusion limitation, and “true” shunt; for this reason, it is also referred as physiologic shunt. In our experience, ECMO support is deemed necessary in patients with an intrapulmonary shunt fraction exceeding 50–60 %, while disconnection is attempted when shunt becomes lower than 40 % (see Fig. 21.4). It is commonly believed that intrapulmonary shunt increases when FiO2 or mixed venous oxygen tension (PvO2) increases, which is exactly what ECMO does. However, a well-designed study on severe ARDS patients undergoing VV ECMO examined the specific role of PvO2 and FiO2 and showed that reducing PvO2 by decreasing the FiO2 of the ML caused a dramatic fall in PaO2, but the intrapulmonary shunt fraction did not substantially change; similarly, increasing the ventilator FiO2 from 60 to 100 % did not modify the intrapulmonary shunt [20]. These results, partially in contrast with other literature data, may indicate that in those ARDS patients, the hypoxic vasoconstriction was blunted.
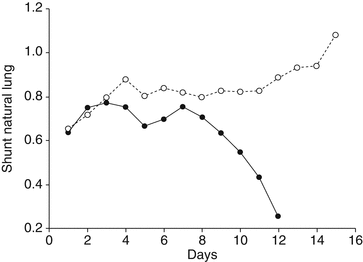
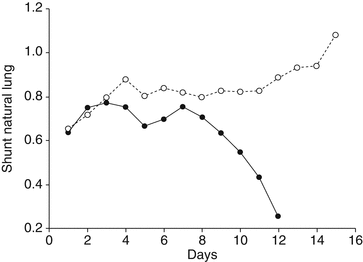
Fig. 21.4
Intrapulmonary shunt of the same two patients reported in Fig. 21.3. Solid circles: the NL shunt starts to improve after 8 days of ECMO support, and the patient was successfully disconnected from the ECMO when NL shunt was lower than 30 %. Empty circles: the patient died during ECMO; after 12 days, the natural lung function worsened and eventually the shunt was 100 %
21.3 Carbon Dioxide
Also when analyzing arterial PCO2 and pH, we need to consider the relative role of NL and ML. Extracorporeal CO2 removal, differently from extracorporeal oxygenation, which mainly depends on extracorporeal blood flow, is primarily related to sweep gas flow: therefore, V’O2 and V’CO2 are independent and need to be measured separately. A decrease in sweep gas flow reduces the artificial lung CO2 extraction (nonlinearly; see Fig. 21.5 panel a), and consequently if the ventilation of the natural lung is not adjusted, PaCO2 will increase. In a spontaneously breathing patient, a reduction of the sweep gas flow will be associated with an increased respiratory drive, leading to an increase in minute ventilation and V’CO2NL (Fig. 21.5, panels a and b). In the patient shown in Fig. 21.5, the progressive reduction of sweep gas flow from 6 to 0.5 l/min (and of V’CO2ML from 150 to 40 ml/min) was associated with increased spontaneous patient’s efforts, which may enhance lung recruitment and actually resulted in a higher PaO2 (panel c). However, when the gas flow was further reduced, the patient’s respiratory efforts became excessive, leading both to a dangerous elevation of plateau pressures (panels b and d) and to a not endurable increase of work of breathing and total CO2 production (panel a). Furthermore, the higher respiratory muscles O2 consumption and the concurrent reduction of the extracorporeal support caused a critical decrease in venous oxygen saturation and ultimately a drop in PaO2 (panel c). This example strongly supports the need for a global assessment of the patient, with simultaneous evaluation of gas exchanges (PaO2, PaCO2) and of the ventilatory “load” imposed to the native lung (P plat, minute ventilation). Monitoring the behavior to progressive re-loading of the native lung is helpful in indicating whether or not, and in the case how much, the patient is still dependent on the extracorporeal support (see Chap. 27 for further information on weaning from VV ECMO).
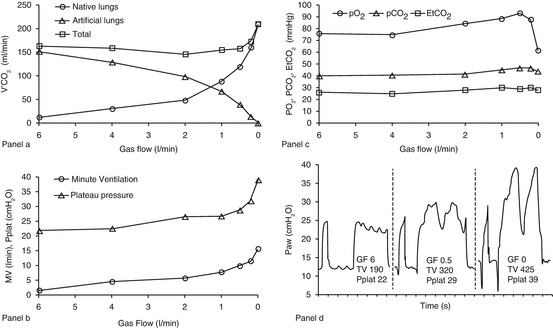
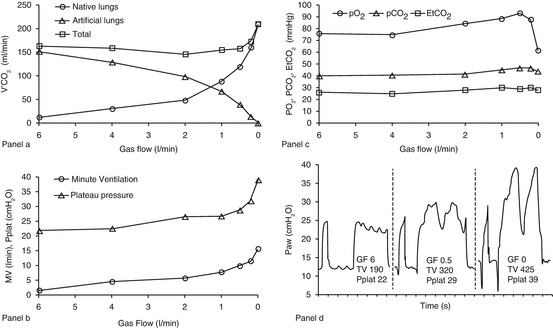
Fig. 21.5
Example of VV ECMO patient’s global assessment. Relationship between native and extracorporeal V’CO2 (panel a), plateau pressure, minute ventilation (panel b), arterial and exhaled gases (panel c) and airways pressure waveforms (panel d) during a progressive reduction of sweep gas flow of the membrane lung in a patient undergoing VV ECMO (BF 3.5 l/min) on spontaneous ventilation (mode: pressure support, support level 12 cmH2O, PEEP 12 cmH2O, FIO2 55 %). In panel d, GF and TV refer to the sweep gas flow (l/min) of artificial ML and to the tidal volume (ml) of NL, respectively
21.4 Respiratory Mechanics
The elastic characteristics of the respiratory system are described by the compliance of the respiratory system (CplRS, ml/cmH2O) which is the change in volume (ΔV) for any applied pressure variation (ΔP):
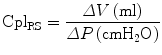
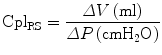
A normal value of CplRS in healthy adults is around 100 mL/cmH2O, but during invasive mechanical ventilation, the expected normal value is lower, around 50–60 mL/cmH2O [21], and it further decreases down to 30–40 mL/cmH2O or less in patients with ALI/ARDS [21, 22].
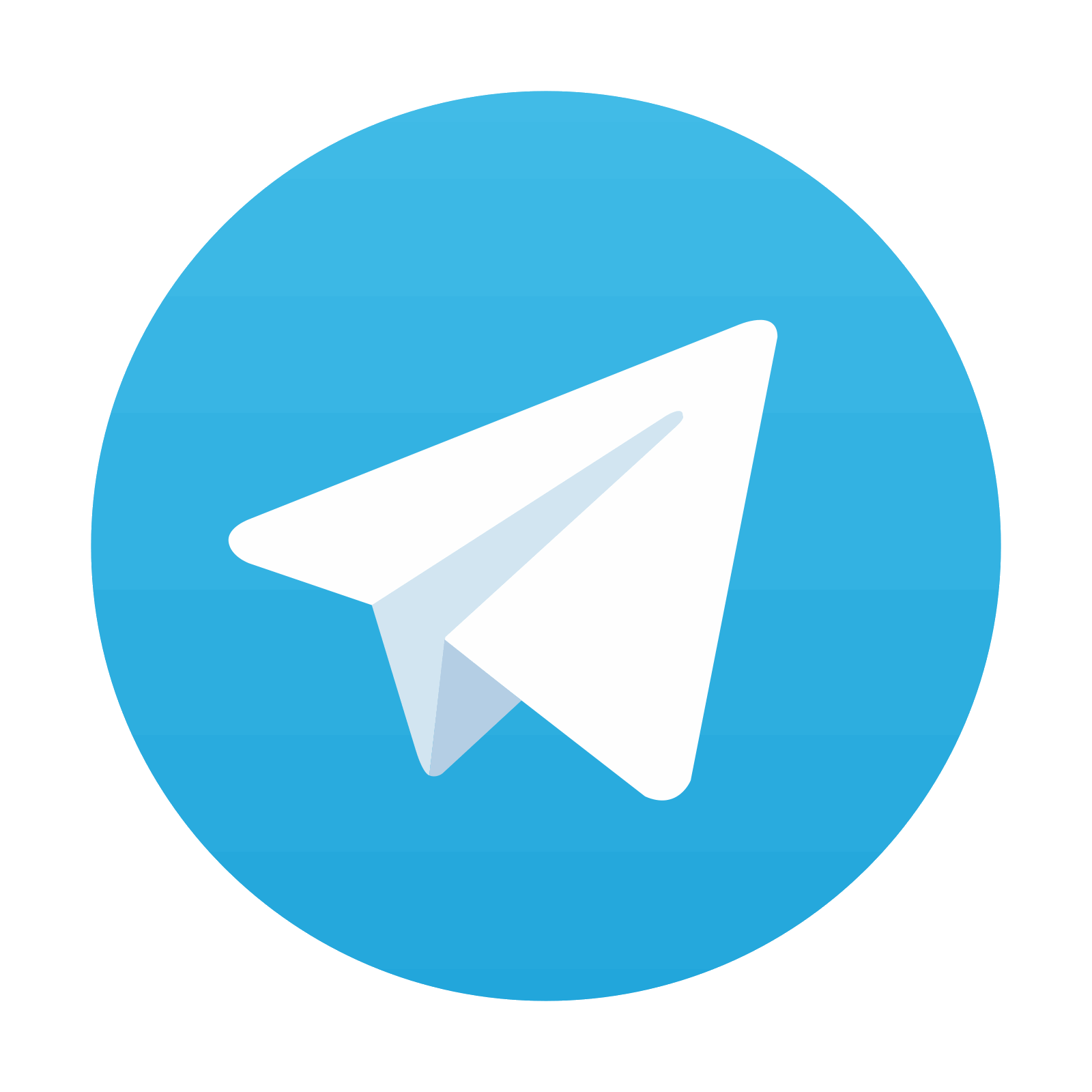
Stay updated, free articles. Join our Telegram channel
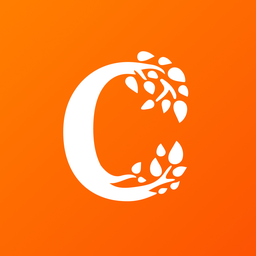
Full access? Get Clinical Tree
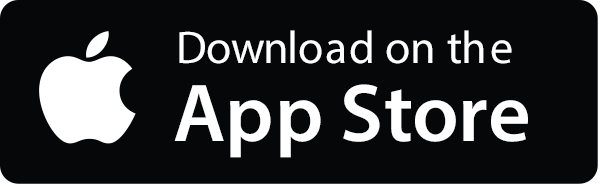
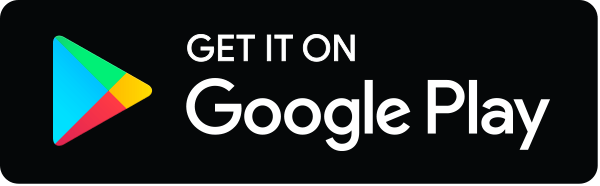