We studied the spatial relations among hyperemic myocardial blood flow (hMBF), contractile function, and morphologic tissue alterations in 19 patients with hypertrophic cardiomyopathy (HC). All patients were studied with oxygen-15 water positron emission tomography during rest and adenosine administration to assess myocardial perfusion. Cardiovascular magnetic resonance was performed to derive delayed contrast-enhanced images and to calculate contractile function (E cc ) with tissue tagging. Eleven healthy subjects underwent similar positron emission tomographic and cardiovascular magnetic resonance scanning protocols and served as a control group. In the HC group, hMBF averaged 2.46 ± 0.91 ml/min/g and mean E cc was −14.7 ± 3.4%, which were decreased compared to the control group (3.97 ± 1.48 ml/min/g and −17.7 ± 3.2%, respectively, p <0.001 for the 2 comparisons). Delayed contrast enhancement (DCE) was present only in patients with HC, averaging 6.2 ± 10.3% of left ventricular mass. In the HC group, E cc and DCE in the septum (−13.7 ± 3.6% and 10.2 ± 13.6%) significantly differed from the lateral wall (−16.0 ± 2.8% and 2.4 ± 5.9%, p <0.001 for the 2 comparisons). In general, hMBF and E cc were decreased in segments displaying DCE compared to nonenhanced segments (p <0.001 for the comparisons). In the HC group, univariate analysis revealed relations of hMBF to E cc (r = −0.45, p <0.001) and DCE (r = −0.31, p <0.001). Multivariate analysis revealed that E cc was independently related to hMBF (beta −0.37, p <0.001) and DCE (beta 0.28, p <0.001). In conclusion, in HC hMBF is impaired and related to contractile function independent from presence of DCE. When present, DCE reflected a progressed disease state as characterized by an increased perfusion deficit and contractile dysfunction.
Microvascular dysfunction is a common feature in patients with hypertrophic cardiomyopathy (HC), despite angiographically normal coronary artery anatomy. Consequently, hyperemic myocardial blood flow (hMBF) is hampered. It has been postulated that microvascular dysfunction predisposes to myocardial ischemia, resulting in contractile dysfunction and scar formation. Indeed, hMBF measured with first-pass cardiovascular magnetic resonance imaging or nitrogen-13 ammonia positron emission tomography has been related to wall thickening and delayed contrast-enhanced cardiovascular magnetic resonance imaging. However, scar tissue affects the kinetics of gadolinium and uptake of nitrogen-13 ammonia, and thus estimates of perfusion. In addition, systolic wall thickening has limited accuracy as a marker of contractile function in the presence of left ventricular (LV) hypertrophy. Oxygen-15–labeled water positron emission tomography, however, determines perfusion in perfusable tissue only, excluding scar tissue from its MBF estimates. In addition, cardiovascular magnetic resonance tissue tagging yields data on myocardial systolic deformation independent from wall thickness, thus serving as a more reliable index of contraction. Therefore, the present study was conducted to investigate inter-relations in hMBF, regional contractile function, and myocardial injury using oxygen-15–labeled water positron emission tomographic versus cardiovascular magnetic resonance tissue tagging and delayed contrast enhancement (DCE).
Methods
Nineteen patients with symptomatic HC were enrolled in the study. HC was diagnosed according to presence of LV septal hypertrophy on 2-dimensional echocardiography (maximal wall thickness >15 mm in adults or >13 mm in relatives of a patient with HC) in the absence of any other systemic or cardiac disease. All patients exhibited asymmetrical LV hypertrophy at the interventricular septum. All patients underwent coronary angiography to exclude presence of coronary artery disease. Use of medication at time of enrollment was not discontinued during the study. Eleven healthy volunteers with normal electrocardiogram, normal physical examination, and without a relevant medical history were included as a control group. The study protocol was approved by the medical ethics review committee of the VU University Medical Center, Amsterdam, The Netherlands.
All positron emission tomographic scans were acquired using an ECAT EXACT HR+ scanner (Siemens/CTI, Knoxville, Tennessee) in 2-dimensional mode. All patients were monitored with single-lead electrocardiography and blood pressure and heart rate were recorded at regular intervals during positron emission tomographic studies. A transmission scan was performed using 3 rotating rod sources filled with gallium-68/germanium-68 solution. Subsequently, oxygen-15–labeled water 1,100 MBq dissolved in a 5-ml saline solution was injected intravenously, followed by a 40-ml saline solution flush at a rate of 4 ml/s. A dynamic emission scan was acquired, consisting of 40 frames with variable frame length for a total time of 10 minutes (12 × 5, 12 × 10, 6 × 20, and 10 × 30 seconds). After the study at rest, MBF was determined during hyperemia by infusion of adenosine at a rate of 140 μg/kg of body weight per minute. Emission data were corrected for physical decay of oxygen-15, dead time, scatter, randoms, and photon attenuation. Cardiovascular magnetic resonance studies were performed in a 1.5-T whole body scanner (Magnetom Sonata, Siemens, Erlangen, Germany) using a 6-channel phased-array body coil. After survey scans, a retro-triggered balanced steady-state free precession gradient-echocardiographic sequence was used for cine imaging. Image parameters were slice thickness 5 mm, slice gap 5 mm, temporal resolution <50 ms, repetition time 3.2 ms, echocardiographic time 1.54 ms, flip angle 60°, and typical image resolution 1.3 × 1.6 mm. Number of phases within the cardiac cycle was set at 20. Four-, 3-, and 2-chamber view cine images were obtained. Then, a stack of 10 to 12 short-axis slices covering the left ventricle was used for assessing LV volumes, mass, and ejection fraction. The method of planning image acquisition for LV coverage has been described previously. Cine images were acquired during 1 breath-hold in mild expiration. Cine imaging with myocardial tagging was applied to create noninvasive markers (tags) within the myocardium for calculation of strain. Five short-axis tagged images with complementary spatial modulation of magnetization tagging for improved strain calculations were acquired as previously described. Delayed contrast-enhanced images were acquired 10 to 15 minutes after intravenous administration of gadolinium 0.2 mmol/kg using a 2-dimensional segmented inversion-recovery prepared gradient-echocardiographic sequence. Inversion-recovery time was 250 to 300 ms.
Data were transferred to a SUN workstation (SUN Microsystems Inc., Knoxville, Tennessee) and analyzed using Siemens/CTI software and an additional tracer kinetic analysis software developed in MATLAB (Mathworks, Natick, Masssachusetts). Reconstruction of oxygen-15–labeled water emission sinograms was performed using filtered back-projection with a Hanning filter at 0.5 of Nyquist frequency, resulting in a transaxial spatial resolution of ≈7 mm full width at 1/2 maximum. Regions of interest were defined manually on oxygen-15–labeled water short-axis summed-uptake images at basal, midventricular, and apical levels of the left ventricle according to a 12-segment model as described previously in detail. This set of regions of interest was projected onto dynamic oxygen-15–labeled water images to generate time–activity curves. Additional regions of interest were defined in the left atrial and right ventricular blood pools. This latter set of regions of interest was projected onto the dynamic oxygen-15–labeled water images to generate time–activity curves of intravascular activity. These time–activity curves were used as image-derived input functions and for use in spillover correction of myocardial tissue time–activity curves. Using a standard single tissue compartment model, MBF (milliliters per minute per gram of perfusable tissue) was determined for all myocardial oxygen-15–labeled water time–activity curves. Average MBF was calculated by grouping all regions of interest, whereas regional blood flows were calculated by grouping specific regions of interest. Corrections were made for LV and right ventricular spillover effects using the method described by Hermansen et al. In addition, coronary microvascular resistance at rest was calculated by dividing mean arterial pressure by MBF, whereas minimal coronary microvascular resistance was derived in similar fashion only during hyperemic conditions. LV volume analysis was performed by manually drawing epicardial and endocardial contours on all end-diastolic and end-systolic LV short-axis images. Global LV function parameters including end-diastolic volume, end-systolic volume, ejection fraction, and myocardial mass were then derived from cine images using MASS software (MEDIS, Leiden, The Netherlands). Tagging images were used to generate circumferential strain curves for each myocardial segment. Subsequently, maximum contractile function (E cc ) was derived for each segment from the strain curves. Similar segmentation for average and regional analyses of E cc was used as described for positron emission tomographic data. Because circumferential shortening is determined by shortening of myofibers, E cc is expressed as a negative value. Each myocardial segment was evaluated for presence of hyperenhancement, which was defined as an area of signal enhancement >5 SD of the signal of nonenhanced myocardium. Extent of DCE was expressed as percent total myocardial tissue area studied. Similar segmentation for average and regional analyses of myocardial hyperenhancement was used as described for positron emission tomographic data.
Results are presented as mean ± SD. For comparison of 2 datasets, unpaired Student’s t test was used. For comparison of multiple datasets, 1-way analysis of variance was applied with post hoc Bonferroni adjustment for inequality. Correlations between variables were evaluated with univariate and multivariate analyses. All tests were performed 2-sided and a p value <0.05 was considered statistically significant.
Results
Baseline characteristics of the HC and control study populations are presented in Table 1 . All patients HC except 4, in whom side effects were considered intolerable, used β-receptor blockers and/or calcium channel blockers, the subtypes and dosages of which are specified in Table 2 . Hemodynamic parameters obtained during at-rest and hyperemic positron emission tomographic studies are listed in Table 3 .
Characteristic | HC | Control | p Value |
---|---|---|---|
(n = 19) | (n = 11) | ||
Men/women | 11/8 | 7/4 | 0.77 |
Age (years) | 55 ± 14 | 53 ± 3 | 0.86 |
Body surface area (m 2 ) | 2.1 ± 0.2 | 2.0 ± 0.2 | 0.72 |
Left ventricular mass (g) | 178 ± 58 | 99 ± 21 | <0.001 |
Left ventricular end-diastolic volume (ml) | 191 ± 33 | 179 ± 33 | 0.80 |
Left ventricular end-systolic volume (ml) | 76 ± 20 | 73 ± 21 | 0.72 |
Left ventricular ejection fraction (%) | 59 ± 8 | 61 ± 5 | 0.98 |
Left ventricular outflow tract obstruction (>30 mm Hg) | 5 (26%) | 0 | <0.001 |
NH 2 -terminal pro–brain natriuretic peptide (ng/L) | 535 ± 536 | 61 ± 53 | <0.001 |
Medication | Patients (%) |
---|---|
None | 4 (21%) |
Metoprolol 100 mg | 4 (21%) |
Metoprolol 50 mg | 2 (11%) |
Bisoprolol 2.5 mg | 2 (11%) |
Metoprolol 50 mg, diltiazem 300 mg | 2 (11%) |
Bisoprolol 2.5 mg, verapamil 180 mg | 2 (11%) |
Metoprolol 100 mg, verapamil 80 mg | 1 (5%) |
Verapamil 240 mg | 1 (5%) |
Diltiazem 200 mg | 1 (5%) |
At Rest | Hyperemia | |||||
---|---|---|---|---|---|---|
HC | Control | p Value | HC | Control | p Value | |
Systolic blood pressure (mm Hg) | 130 ± 25 | 124 ± 16 | 0.48 | 112 ± 19 | 122 ± 12 | 0.13 |
Diastolic blood pressure (mm Hg) | 75 ± 9 | 73 ± 8 | 0.55 | 64 ± 8 | 65 ± 8 | 0.74 |
Mean arterial pressure (mm Hg) | 94 ± 13 | 90 ± 10 | 0.52 | 80 ± 10 | 84 ± 8 | 0.27 |
Heart rate (beats/min) | 61 ± 9 | 66 ± 11 | 0.19 | 87 ± 14 | 95 ± 15 | 0.15 |
Table 4 presents regional at-rest and hMBF, E cc , and DCE for the 2 study groups. In total 228 segments were analyzed in the HC group and 132 in the control group (12 segments per subject). In the HC group, MBF at rest averaged 0.96 ± 0.21 ml/min/g and was slightly lower compared to the control group (1.09 ± 0.36 ml/min/g, p = 0.10). MBF was significantly decreased in the hypertrophied septum and inferior wall compared to the lateral wall (p <0.01 and p <0.05, respectively). Hyperemic MBF in the HC group averaged 2.46 ± 0.91 ml/min/g, which was significantly lower compared to the control group (3.97 ± 1.48 ml/min/g, p <0.001). In the 2 study groups, hMBF was distributed homogenously across all regions. E cc in the HC group averaged −14.7 ± 3.4%, which was significantly lower compared to controls (−17.7 ± 3.2%, p <0.001). In addition, E cc was distributed more heterogenously in HC, with significant impairment of E cc in the hypertrophied septum compared to the lateral wall (p <0.001). On average, 6 ± 10% of the myocardium displayed DCE in HC, predominantly localized in the basal anteroseptal region at the junction with the right ventricular free wall (14 ± 12%). Mean end-diastolic wall thickness of the hypertrophied septum was 16.3 ± 2.8 mm. Analysis of all myocardial HC segments revealed that hMBF decreased in proportion to the increase in end-diastolic wall thickness (p <0.001, analysis of variance; Figure 1 ). In contrast, extent of DCE increased in proportion to the increase in end-diastolic wall thickness (p <0.001, analysis of variance). During conditions at rest, coronary microvascular resistance was 102 ± 22 mm Hg/ml/min in the HC group, which was not significantly different from the control group (86 ± 19 mm Hg/ml/min, p = 0.07). Coronary microvascular resistance during hyperemic conditions, however, was significantly higher compared to controls (37 ± 15 vs 22 ± 6 mm Hg/ml/min, p = 0.004).
Region | MBF (ml/min/g) | hMBF (ml/min/g) | E cc (%) | DCE (%) | ||||
---|---|---|---|---|---|---|---|---|
HC | Control | HC | Control | HC | Control | HC | Control | |
Anterior | 1.00 ± 0.22 | 1.18 ± 0.32 | 2.36 ± 1.01 | 4.40 ± 1.37 | −14.3 ± 3.3 | −16.9 ± 2.9 | 7.1 ± 10.6 | 0 |
Lateral | 1.02 ± 0.27 ⁎ † | 1.18 ± 0.35 | 2.54 ± 0.99 | 3.73 ± 1.30 | −16.0 ± 2.8 | −18.8 ± 3.2 | 2.4 ± 5.9 | 0 |
Inferior | 0.86 ± 0.24 | 0.96 ± 0.30 | 2.43 ± 1.23 | 4.37 ± 2.02 | −14.5 ± 3.4 | −17.5 ± 3.4 | 4.5 ± 5.5 ⁎ | 0 |
Septal | 0.90 ± 0.24 | 1.05 ± 0.40 | 2.34 ± 1.02 | 3.80 ± 1.35 | −13.7 ± 3.6 ‡ | −17.1 ± 3.2 | 10.2 ± 13.6 ‡ | 0 |
Average | 0.96 ± 0.21 | 1.09 ± 0.36 | 2.46 ± 0.91 | 3.97 ± 1.48 | −14.7 ± 3.4 | −17.7 ± 3.2 | 6.2 ± 10.3 | 0 |
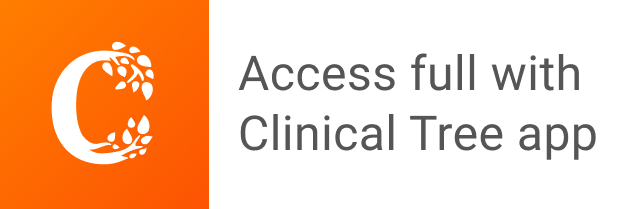