CHAPTER 102 Regenerative Cell-Based Therapy for the Treatment of Cardiac Disease
Despite considerable advances in cardiovascular therapeutics (e.g., interventional revascularization, surgical ventricular restoration, heart transplantation) over the past few decades, our ability to effectively treat ischemic heart disease and congestive heart failure remains limited because necrotic or scarred myocardium cannot be restored. Since 1994, an experimental treatment, called either cardiac cell therapy or cell transplantation, has emerged1; it is an attempt to mimic natural processes that occur during growth and development—namely, the formation of new blood vessels and new myocardial contractile units.2,3 The new cells are expected to replace the damaged ones—thus, the umbrella term regenerative medicine—and effectively restore perfusion and function to the heart.4–6
Early reports from animal and clinical investigations disagree on the extent of physiologic myocardial renewal in adults, but evidence suggests that new cardiomyocytes are indeed generated in low numbers in the postnatal heart,7 contrary to the original belief that it was entirely a postmitotic organ. On the basis of postmortem histologic analysis in mice and other animals, it is proposed that renewal is achieved by stem cells that infiltrate normal and infarcted myocardium.8 Animal studies have also showed that bone marrow stem cells9 or skeletal myoblasts10 transplanted into a damaged heart might generate cardiomyocytes and successfully repopulate the heart tissue. Furthermore, multipotent stem cells or progenitor cells can induce new blood vessel formation in the damaged myocardium, as well as the proliferation of existing vasculature.11,12 Growing evidence from clinical trials has indicated that stem cell or progenitor cell therapy can restore the perfusion of and improve the function of ischemic or infarcted myocardium in humans.13,14 This has led to even greater interest in cell-replacement strategies, with the goal of safely and effectively restoring perfusion and contractility to ischemic, stunned, hibernating, or scarred myocardium.15
CELL TYPES AND THEIR ISOLATION
An ideal cell population for repairing the damaged heart should be readily available, be capable of myogenesis and neovascularization, positively affect regional and global ventricular remodeling, and not lead to unwanted side effects. Nine cell populations have so far been used in animal models, and several of them are also being tested clinically (Table 102-1 and Fig. 102-1).
Skeletal Myoblasts
Cell-based cardiac repair began with the transplantation of autologous skeletal myoblasts or satellite cells that reside in skeletal muscle, which are considered stem cells based on their ability for self-renewal and differentiation. Myoblast and muscle progenitor cells are CD56 positive, desmin positive, and CD45 negative.16 They can be obtained from a skeletal muscle biopsy under local anesthesia, are easily harvested and expanded in vitro, and offer a good survival rate. In animal studies, after cardiac injury, transplanted skeletal myoblasts are able to differentiate into myotubes, retain muscle properties, and improve cardiac function.17,18 Myoblast transplantation has been shown to augment systolic and diastolic performance in animal models, possibly via a paracrine effect.19 The potential for autologous use makes them a clinically feasible therapy. Some clinical case reports suggest that myoblast therapy may improve clinical symptoms and ventricular function16,20,21; however, the transplanted myoblasts remain committed to a skeletal muscle fate, do not differentiate into cardiomyocytes,22 and lack the adhesion and gap junction proteins necessary to electrically couple the cells to the surrounding myocardium, thus posing a risk of arrhythmia.23
Embryonic Stem Cells
Embryonic stem cells (ESCs) are the prototypical stem cells that fulfill all of the criteria of a stem cell: clonality, self-renewal, and pluripotency. ESCs are obtained from the blastocyst inner cell mass, and they can differentiate into all cell types present in the adult. Human ESCs were successfully isolated in 1998,24 and these cells can be differentiated into cardiomyocytes.25,26 Undifferentiated human ESCs express the stem-cell markers Oct4, stage-specific mouse embryonic antigen (SSEA)3/4, TRA-1-60, and TRA-1-81.27 Some studies demonstrated that ESCs can express cardiac-specific genes and spontaneously beat with chronotropic responsiveness to various drugs in vitro.28 Other studies indicated that ESCs can remain viable in the myocardium and over time become more organized into structures that resemble intercalated discs.29 Transplantation of ESC-derived cardiomyocytes has led to improvement in ventricular function in several rodent infarct models.30 Because ESCs also have the ability to form endothelial and smooth muscle cells, they may possess the added advantage of being able to provide the supporting tissue (e.g., blood vessels) needed to maintain the newly developed cardiomyocytes. However, to date, no clinical trials have been initiated using human ESCs, not only because of the ethical challenges of these cells but also because of concerns of tumorigenicity, ectopic differentiation, and immunogenicity.31 Nevertheless, the study of ESC differentiation into cardiomyocytes could lead to a better understanding of the events required for other cells to successfully differentiate into cardiomyocytes, as well.
Bone Marrow–Derived Stem Cells
The bone marrow is a rich reservoir of tissue-specific stem and progenitor cells. Bone marrow cells may serve as a central repository for the primitive stem cells that have the capacity to repopulate somatic tissues; therefore, most recent studies of stem cell–based therapy have focused on bone marrow–derived cell populations.8 However, the bone marrow cells (BMCs) used in a majority of cardiac cell therapy trials (Table 102-2) contained only a small fraction of actual stem or progenitor cells, the majority of cells having already committed to hematologic lineage.
Bone marrow can be aspirated under local or general anesthesia. Either the entire mononuclear cell fraction is obtained or specific subpopulations are purified, and isolated cells can be injected into the heart without need of further ex vivo expansion.32 Because of its autologous nature, related clinical precedents in the bone marrow transplant field, and simplicity, bone marrow is, at present, the most frequently used source of cells for clinical cardiac cell therapy. Bone marrow contains a complex assortment of progenitor cells, including hematopoietic stem cells (HSCs), endothelial progenitor cells (EPCs), and mesenchymal stem cells (MSCs).
Hematopoietic Stem Cells
HSCs are stem cells that give rise to all the blood cell types. HSCs can be obtained directly from the bone marrow of adults, or from the blood after pretreatment with cytokines, such as granulocyte colony-stimulating factors (G-CSFs), which induce cells to be released from the bone marrow compartment. Generally, HSCs are identified by their small size, their lack of lineage (lin) markers, and the presence of various antigenic markers on their surface, such as CD34, CD38, CD90, CD133, CD105, and CD45, and also c-kit (CD117).33
Because of their regeneration capacities, HSCs have been used in cell cardiac therapy. It was initially reported that lin− and c-kit+ HSCs differentiated into cardiomyocytes, endothelial cells, and smooth muscle cells when injected into infarcted mouse myocardium, and that they improved ventricular function.9 Clinical trials also showed that intracoronary infusion of peripheral blood HSCs following G-CSF administration improved cardiac function and promoted angiogenesis in patients with myocardial infarction.34,35 However, recently, the whole concept of HSC plasticity has been questioned by experiments that demonstrate that GFP+ HSCs injected into nontransgenic recipients reconstitute only peripheral blood leukocytes and do not contribute appreciably to nonhematopoietic tissues.36
Endothelial Progenitor Cells
EPCs are a specialized subset of hematopoietic cells found in the bone marrow, vascular wall, and peripheral circulation.37 With their varied sources of procurement, derivation, and methods of culture, EPCs share expression of cell-surface markers that may or may not include CD31, CD34, CD133 (AC133), c-kit, and other endothelial markers, including vascular endothelial growth factor (VEGF)-R2 and VE-cadherin (Fig.102-2B).38 CD133 and CD34 are often used as markers for selection and purification of EPCs, because CD133 is not found on mature endothelial cells, and CD34 is not found on the undifferentiated stem cells from which endothelial progenitor cells are derived.39 For clinical use, one often used, heterogeneous EPC population may be selected by the ex vivo culture of mononuclear blood cells in “endothelium-specific” medium for 3 to 7 days.
Experimentally, circulating EPCs have been shown to be mobilized endogenously in response to tissue ischemia, after which they can augment the neovascularization of ischemic tissues.40 Administration of EPCs in animal models of cardiac ischemia resulted in increased blood vessel formation, decreased infarcted size, and improved ventricular function.41 Other observations have shown that EPCs prevented cardiomyocyte apoptosis, reduced ventricular remodeling, and improved cardiac function in areas of neovascularized ischemic myocardium.42 The ability of human EPCs to transdifferentiate into cardiomyocytes has also been reported,43 but not all studies support this point.44 Furthermore, studies of human EPCs have shown that they secrete pro-angiogenic factors45 and that EPC function is impaired in the setting of diabetes or heart failure, as well as after exposure to cardiopulmonary bypass in patients undergoing coronary revascularization.11 However, whether these cells actually incorporate into new vessels or enhance the endogenous angiogenic response through paracrine effects remains unclear. Although no large-sample-size clinical trials to date have evaluated their effects in patients, EPCs represent a promising and novel strategy to induce therapeutic angiogenesis.46
Mesenchymal Stem Cells
MSCs are another subpopulation of bone marrow–derived stem cells, and they appear to have in vitro multilineage differentiation capacity. Unlike HSCs and EPCs, MSCs are negative for the surface expression of both CD34 and CD45.47 In culture, expanded and adherent MSCs are positive for SH2, SH3, CD29, CD44, CD71, CD90, CD106, CD120a, and CD124 (Fig.102-2A).48 However, there is no test that can be performed on a single cell to determine whether that cell is an MSC. Therefore, these surface antigens can be used to isolate a population of cells that have similar self-renewal and differentiation capacities, yet MSCs, as a population, typically do not all express the proposed markers.
Some studies demonstrated that purified human MSCs adopt a cardiomyocytic phenotype and may attenuate contractile dysfunction and prevent adverse remodeling in murine and swine models of myocardial infarction.48,49 Some clinical trials have also shown results similar to those seen in animal studies.50 These cells might secrete pro-angiogenic growth factors and cytokines and, therefore, have been targeted for myocardial repair.51–53 A major potential advantage of using MSCs for cell therapy is that they appear to have reduced immunogenicity, at least initially, which might allow allogenic use.5,54 In addition, MSCs are relatively easy to expand and maintain ex vivo. This would offer the benefit of obtaining cells from young, healthy donors, and such cells might have enhanced stem cell activity compared with cells from older patients with comorbidities. However, others have shown conflicting results and have suggested that MSCs may not transdifferentiate to cardiomyocytes and that we need to better understand the effects of MSCs on myocardial injury. Because MSCs are larger cells and under normal circumstances cannot traverse a capillary bed, they are less suitable for intravenous injection.
Adipose-Derived Stem Cells
Adipose tissue is an additional source of distinct subsets of stem or progenitor cells potentially useful for cardiac repair and neovascularization.55 Both MSCs and EPCs have been isolated after enzymatic digestion of adipose tissue, and they showed beneficial effects in small animal studies.56 Adipose tissue is less expensive to acquire than other source tissues, the operation is less invasive, and greater quantities are available. Although the cells are relatively rare at the beginning, clinically relevant stem cell numbers can be extracted from isolated adipose tissue because its stem cell proliferation rate is higher than that of bone marrow–derived MSCs.57 Therefore, adipose tissue is an abundant, practical, and appealing source of donor tissue for autologous cell therapy. The therapeutic benefit of adipose tissue–derived cells is currently being studied, and techniques to isolate and purify the small number of potent cells will need to be optimized if clinical use is contemplated.
Side Population Cells
Side population (SP) cells, defined by their ability to strongly efflux Hoechst 33342 fluorescence dye, are an attractive candidate among somatic stem cells. SP cells are a rare cell population detected in a variety of tissues including bone marrow,58 embryoblasts,59 the peripheral circulation, and the adult heart60 (see Fig. 102-1). SP cells can be isolated by dual-wavelength flow cytometry because of their capacity to efflux the Hoechst dye. Surface marker analysis shows that SP cells express high levels of CD45, CD59, CD43, CD49d, CD31, and integrin markers (α6-integrin, β1-integrin, Sca-1, keratin 14, and keratin 19), but that they lack CD34, and CD71.61,62
An increasing number of studies shows the multipotential capacity of SP cells isolated from various tissues. SP cells isolated from murine adult bone marrow exhibit potent HSC activity when compared with main-population cells. Furthermore, SP cells derived from murine skeletal muscle exhibit both hematopoietic and myogenic potential in vivo.62 Additionally, when cultured SP cells from neonatal rat hearts were treated with oxytocin or trichostatin A, some expressed cardiac-specific genes and proteins and showed spontaneous beating. When intravenously infused into adult rats, many more of these cardiac SP (CSP) cells homed to the injured heart than to normal heart. CSP cells in injured heart tissue differentiated into cardiomyocytes, endothelial cells, or smooth muscle cells.63
Induced Pluripotent Stem Cells
Induced pluripotent stem (iPS) cells are artificially derived from a non-pluripotent cell, typically an adult somatic cell, by inducing “forced” expression of certain genes. Human iPS cells are believed to be identical to natural pluripotent stem cells (e.g., human ESCs) in many respects, such as morphology, proliferation, surface antigens, gene expression, epigenetic status of pluripotent cell-specific genes, and telomerase activity. Furthermore, these cells can differentiate into cell types of the three germ layers in vitro and might form teratomas. However, the full extent of their relationship to natural pluripotent stem cells and their preclinical applicability for cardiac regeneration are still being assessed.64
Recent studies have demonstrated that iPS cells can be generated from adult human cells, such as skin fibroblasts. Pluripotency is induced via the introduction of a quartet of genes, such as OCT4 and SOX2 in combination with either KLF4 and c-MYC65–68 or LIN28 and NANOG.64,67,69 One study showed that iPS cells generated from human adult somatic cells can differentiate into cardiac myocytes in vitro. These differentiated cells expressed cardiomyocyte markers and were observed to beat spontaneously, whereas the expression of OCT3/4, SOX2, and NANOG markedly decreased.65,70 Although these cells have yet to be tested in cardiac cell therapy models in vivo, they may be a cell of choice, offering the additional benefit of individual patient-specific cells.71
Cardiac Stem Cells
It was originally thought that heart was incapable of regeneration or repair, but recently it was reported that the mammalian myocardium includes a small proportion of stem cells that express cell-surface markers c-kit, Sca-1, and Isl-1.72 The discovery of cardiac stem cells (CSCs) offers the potential for in vivo induction of proliferation and differentiation of these cells, which are poised to acquire a cardiac phenotype and, therefore, might be optimally suited for cardiac repair. CSCs can be isolated and expanded from human myocardium obtained by an endovascular biopsy procedure; however, they possess an extremely low rate of myocardial cell turnover.73 Recently, several groups have reported that CSCs can generate cardiomyocytes or vascular lineages (or both) in vitro and in vivo.72,74 Cardiosphere-derived CSCs, as well as c-Kit+ CSCs, are capable of long-term self-renewal and can differentiate into the major specialized cell types of the heart (i.e., myocytes, endothelial cells75 and smooth muscle cells). The exact origin of these c-Kit+, or cardiosphere-derived CSCs and the mechanisms maintaining the CSC pool remain unclear. Recent studies suggest that c-Kit+ and cardiac progenitor cells may be from the bone marrow76,77; however, these studies cannot entirely exclude the possibility that specific subpopulations of CSCs originated from the heart and that these CSCs may represent remnants from embryonic development in selected niches in the heart.78 Although CSCs can be clonally expanded and have minimal risk of immune rejection or teratoma formation in autologous use, no clinical data are available yet, as these cells have not been completely and consistently characterized, and their physiologic role in myocardial repair and regeneration after injury remains unclear.
In summary, several different populations of adult stem cells have been identified and used for improving cardiac function after myocardial ischemia and infarction, but it is uncertain which of these cells have the greatest therapeutic potential. Although marrow–derived progenitor cell therapy has thus far proved safe and probably beneficial, the cells’ intrinsic regeneration potential is controversial. CSCs have the potential to be patient specific, but isolation and culture procedures are in the early stages of development. ESCs have the greatest differentiation potential but face ethical barriers and have the greatest risk for teratoma formation. In addition, whether ESC derivatives will be rejected by the host immune response is still under debate.79 As the field develops, it appears clear that a ranking of candidate cells for cell therapy will be based not only on the assessment of the functional capacities of the cells but also on the safety and feasibility of treatment in the clinical setting.
DELIVERY METHODS FOR CELL TRANSPLANTATION
Cellular therapy involves delivering stem cells to the target area. Generally, cells can be injected intravenously, into coronary arteries, or into the ventricular wall via a percutaneous transendocardial or a surgical transepicardial approach (Fig. 102-3). Each approach has advantages and disadvantages (Table 102-3).
Table 102–3 Administration Methods
Route | Advantages | Disadvantages |
---|---|---|
Intravenous injection | Simplest | Few migrate into the target |
Intracoronary injection | Cells can travel directly into myocardial regions in which nutrient blood flow and oxygen supply are preserved; low risk for arrhythmia | Unsuitable for larger stem cells, hinder cellular transmigration, exacerbation of atherosclerosis |
Intramyocardial injection | Most reliable under direct visualization | Invasive, less suitable for acute myocardial infarction |
Intravenous Injections
Intravenous injection is the simplest method of cell administration. However, delivery is nonspecific, and a great degree of dependence on homing of the stem cells is required for them to reach the myocardium. Intravenously injected cells may also become trapped in other organs, particularly in lymphoid tissues, so only a small portion enters the coronary circulation and migrates into ischemic myocardium.80,81 Additionally, the larger cell types such as MSCs or skeletal myoblasts may not penetrate beyond a given capillary bed, making these cells unlikely to reach their myocardial target using intravenous injection.
Intracoronary Injections
Arterial intracoronary injection can be performed at the time of selective coronary angiography, and it is well suited for the delivery of cells to a specific coronary territory because of the segmental nature of coronary artery disease. The advantage of intracoronary infusion is that cells can travel directly into the myocardial regions where nutrient blood flow and oxygen supply are preserved, which ensures a favorable environment for cell survival, a prerequisite for stable engraftment.32,82 Indeed, in a recent clinical study, homing of unselected bone marrow cells to the infarct region was observed only after intracoronary delivery but not after intravenous infusion.80 However, high coronary flow after stem cell injection may prevent stem cells from adhering to the myocardial capillaries and thus hinder cellular transmigration into the infarcted zone. Furthermore, atherosclerotic plaque inherently harbors potent angiogenic processes, and it is plausible that stem cell injections in a disease segment could exacerbate macrovascular as well as microvascular coronary disease. Finally, this technique may not be suitable for certain types of larger stem cells, such as skeletal myoblasts and MSCs, which may be prone to embolization. Despite these theoretical issues, intracoronary injection of stem cells has been the preferred route in most clinical studies.
Intramyocardial Injection
Electromechanical mapping (e.g., using the NOGA system [Biosense-Webster, Haifa, Israel]) may make surgical transepicardial injection more reliable, or it may be used to help perform transendocardial injection as a percutaneous procedure. NOGA has been in existence for 8 years,83 but its popularity has not increased as much as was expected.
Intramyocardial injections are the most specific route for cell injection; however, they are still not entirely specific, and some cell spillage does occur. Giving multiple injections of concentrated cell suspensions at minimal volumes appears to be preferable to giving less frequent injections of larger volumes. This method is most reliable and feasible, and the cells may reach all areas of the myocardium.32 Nevertheless, it is difficult to predict the survival and function of progenitor cells injected into uniformly necrotic tissue. This is because the infarct territory may lack living cells and therefore may not furnish the necessary instructive signals or blood flow for the delivery of oxygen and nutrients needed for transplanted cell engraftment and differentiation. Additionally, in diffuse diseases such as dilated nonischemic cardiomyopathy, focal deposits of directly injected cells might be poorly matched to the underlying anatomy and physiology.32,84
The optimal route for administering stems cells is yet to be determined, and certain factors should be taken into consideration. The strength of homing signals may vary in different clinical situations. In more acutely ischemic scenarios, the stem cells may be administered either peripherally or locally through the circulatory system. When the homing signals may be less intense, as may be the case for chronic ischemia, scar, or nonischemic cardiomyopathies, injection of the cells directly into the cardiac muscle may produce a more favorable outcome.40 Certain stem cells, such as skeletal myoblasts, are best administered by means of direct tissue injection because of the potential for embolization when large numbers of these cells are administered. In recent human trials, intracoronary and intramyocardial approaches seem the most likely to be tested. Numerous phase I clinical trials of myocardial stem and progenitor cell delivery using these two approaches have thus far revealed few serious adverse effects.85
MECHANISMS UNDERLYING THE BENEFITS OF CELL TRANSPLANTATION
A crucial issue in designing more rational cell-based therapy approaches for cardiac disease is to understand the mechanisms by which stem cells or progenitor cells can affect myocardial performance.79,86 A number of studies have suggested that adult stem cells undergo novel patterns of development by a process referred to as transdifferentiation or plasticity.9,87 However, others demonstrated that these processes are not considered to occur in a major mechanistic fashion in vivo with the use of nonembryonic cells.88,89 Alternatively, several investigators have provided evidence that paracrine mechanisms are important. For example, transplanted stem cells can release cytokines that recruit circulating stem cells and that activate resident stem cells, which can then themselves become new blood vessel cells and myocytes.9,76,90 Recently, it was also postulated that a concentration-dependent difference in collagen turnover as a result of myogenic precursor cell (MPC) transplantation may be responsible for most of the beneficial remodeling effects noted after MPC therapy, with complete disappearance of the cells by 8 weeks after injection.91–93
Cell Homing
Although its mechanism is incompletely understood, the homing of stem cells to the injured myocardium is essential, as it concentrates the implanted cells in an environment that may guide their growth and function. Cell homing is a multistep cascade, including the initial recognition and interaction with microvascular endothelium, transmigration through the endothelium, and, finally, migration and invasion of the target tissue.71 The whole process relies on a complex interplay between cytokines, chemokines, adhesion molecules, and extracellular matrix–degrading proteases. The capacity of stem cells to migrate and invade is critical for functional integration even when cells are injected directly into the site of injury.71 Additionally, ischemia and hypoxia increase vascular permeability, enhance the release of chemoattractive factors, and promote the expression of adhesion proteins, which may facilitate the homing process.40
The homing of different types of stem cells to areas of tissue injury may occur via several distinct ways. For example, the initial step of homing of progenitor cells to ischemic tissue involves adhesion of progenitor cells to endothelial cells activated by cytokines and ischemia, followed by the transmigration of the progenitor cells through the endothelial cell monolayer.84,94 Naturally, bone marrow–derived cells that may be involved in cardiac repair might undergo mobilization into peripheral blood, with subsequent homing and engraftment into the target tissue.95,96
It is still unclear what environmental cues initiate mobilization and homing of adult stem cells to normal and injured tissue. Growing evidence shows that stem cell migration may be regulated by some ligand–receptor interactions, such as c-kit and stem cell factor (SCF), stromal cell–derived factor-1 (SDF-1) and CXCR4, G-CSF and the G-CSF receptor, and VEGF and flk-1.8,71 Furthermore, integrins are known to mediate the adhesion of various cells, including hematopoietic stem cells and leukocytes, to extracellular matrix proteins and to endothelial cells.97 Additionally, current studies show that the binding of implanted cells to host matrix elements might be involved in cell homing.98
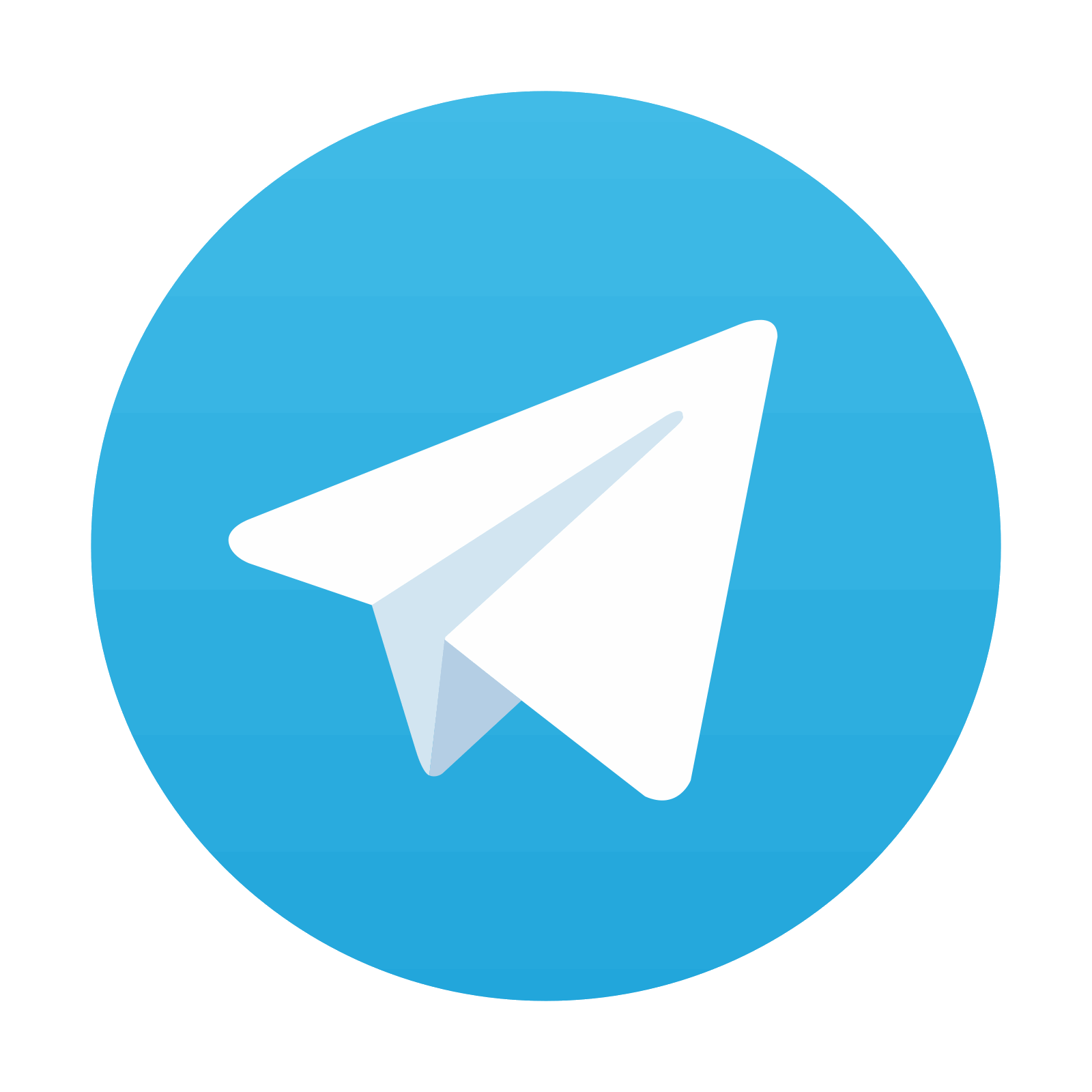
Stay updated, free articles. Join our Telegram channel
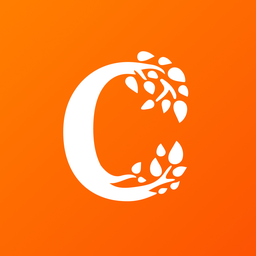
Full access? Get Clinical Tree
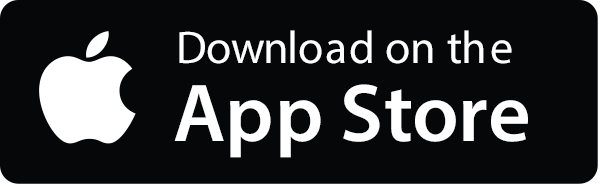
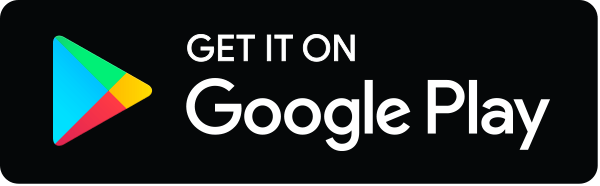