Physiological Principles of Normal Lung Function |
For venous blood to be properly arterialized, the distribution of air and blood within the lung is automatically matched to ensure effective gas exchange across alveolar–capillary membranes. Arterialization comprises a series of interrelated processes that begin with the mechanical performance of the ventilatory apparatus—that is, the lungs and the chest wall, including the rib cage, diaphragm, and abdominal wall. The ventilatory apparatus is critical for replenishing fresh air to the lungs for gas exchange. Although the function of each component of the lung and of the chest bellows can be deranged by injury or disease, the design of the ventilatory apparatus provides for considerable reserve. As a result, mechanical derangements are usually quite severe by the time clinical symptoms appear or arterial blood-gas levels become abnormal.
Depending on the nature of the underlying disorder, assessment of the mechanical properties of the ventilatory apparatus provides several different types of information. In some instances, characterization of the mechanical abnormality provides insight into pathogenesis and affords a quantitative measure of severity. In others, once the nature of the mechanical disorder is understood, the mystery surrounding a life-threatening disorder in gas exchange may be dispelled. Finally, certain breathing patterns make sense only if the mechanical performance of the chest bellows is taken into account.
During breathing, the lungs and chest wall operate in unison. The lungs fill the chest cavity so that the visceral pleura are in contact with the parietal pleura of the chest wall. The two pleural surfaces are separated by only a thin liquid film, which provides the bond holding the lungs and chest wall together.
At the end of a normal exhalation when the respiratory muscles are at rest, the ventilatory apparatus is in a state of mechanical equilibrium. The pressure along the entire tracheobronchial tree from the airway opening to the alveoli is equal to atmospheric pressure. The tendency of the lung is to deflate, however, and lung elastic recoil is directed centripetally. This is counterbalanced by the elastic recoil of the chest wall, which is directed centrifugally to favor an increase in volume. These opposing forces generate a subatmospheric pleural pressure of about –5 cmH2O (Fig. 10-1A). The tendency for the lung to recoil inward and for the chest wall to recoil outward is illustrated by the observation that when the chest is opened at autopsy, the lungs collapse to a nearly airless state and the thorax expands.
Figure 10-1 Respiratory pressures during a breathing cycle. A. End expiration. B. During inspiration. C. End inspiration. Ppl, pleural pressure; PA, pressure in the alveoli; Pao, pressure at the airway opening.
Although it is conventional to consider pleural pressure as a single, mean value that reflects mechanical events within the entire ventilatory apparatus, this is clearly an oversimplification on several accounts: (1) pleural pressure is not directly determinable because normally there is only a potential space between the visceral and parietal pleura; (2) on conceptual grounds, distinctions exist between surface and liquid pleural pressures; (3) pleural pressures are not uniform over the surface of the lungs, being strongly affected by gravity; and (4) transmission of pleural pressures at the surface to alveoli located at different depths and loci with the lungs depends on the structural interplay among supporting structures in the alveolar walls (interdependence), which resists any inclination of individual alveoli or even a lobule to collapse.1 Nonetheless, the concept of mean pleural pressure, as generally used in considerations of respiratory system mechanics, has proved to be of great practical value.2
The contraction of the muscles of inspiration produces the forces that permit the flow of gas along the tracheobronchial tree and the expansion of the lungs and chest. The movement of air into the lungs requires a pressure difference between the airway opening and the alveoli sufficient to overcome the resistance to airflow of the tracheobronchial tree. Also, a pressure difference across the alveolar walls (between the alveoli and pleural space) must be generated to overcome elastic recoil and inflate the lungs. During spontaneous breathing, the action of the inspiratory muscles causes an increased outward pull on the chest wall.3 As a result, the pleural pressure becomes more subatmospheric. This pressure change is transmitted to the interior of the lungs, so alveolar pressure also becomes subatmospheric (Fig. 10-1B). In contrast, during artificial ventilation with a positive-pressure ventilator, a supra-atmospheric pressure applied at the inlet to the airways creates the proper pressure gradient between the airway opening and alveoli for airflow.
Expansion of alveoli depends on the achievement of an appropriate distending pressure across alveolar walls. This distending pressure or transpulmonary pressure is the difference between alveolar (PA) and pleural (Ppl) pressures. As shown in Figure 10-1B, the transpulmonary pressure at end expiration (PA – Ppl) is 5 cmH2O. At the end of inspiration (Fig. 10-1C), the lungs contain more air and the distending pressure which also represents the recoil pressure is greater.
The energy used during inspiration to overcome the elastic resistance of the lungs is stored. Expiration occurs when these forces are released. When the inspiratory muscles relax, the recoil of the lungs causes the alveolar pressure to exceed the pressure at the mouth, and air flows out of the lungs. Although expiration during quiet breathing is passive, the expiratory muscles are engaged at high levels of ventilation to assist the movement of air out of the lungs.
LUNG VOLUMES
The lung volumes and capacities (Table 10-1) are also considered elsewhere in this book (see Appendix B). The end-expiratory position of the lungs, functional residual capacity (FRC), is the major reference point for the subdivisions of lung volume. This position is set by the opposing recoil forces of the lung and chest wall when the respiratory muscles are at rest.
TABLE 10-1 Lung Volumes and Subdivisions
Total lung capacity (TLC), the total volume of air contained in the lungs after a maximal inhalation, is determined by the balance between the force-generating capacity of the inspiratory muscles and the opposing elastic recoil forces of the lung and chest wall.4 Weakness of the muscles of inspiration or increased stiffness of the lung reduces TLC. Loss of retractive forces exerted by the lung, as in emphysema, enlarges TLC.
Residual volume (RV), the volume of air remaining in the lungs after a complete exhalation, is set by the balance between the actions of the expiratory muscle and the recoil forces of the lung, which act to decrease lung volume, and the outward recoil forces of the chest wall, which favor lung expansion. In middle-aged and older individuals, closure of airways at low lung volumes, with air trapping in the lung, is an important determinant of RV.5
STATIC MECHANICAL PROPERTIES OF THE RESPIRATORY SYSTEM
To assess the elastic properties of the ventilatory apparatus, it is expedient to evaluate the elastic properties of the lungs and chest separately. Elastic properties are conventionally assessed over a fixed range of volumes during periods of arrested airflow.
ELASTIC PROPERTIES OF THE LUNGS (PULMONARY COMPLIANCE)
The change in transpulmonary pressure required to effect a given change in the volume of air in the lungs is a measure of the distensibility, or compliance, of the lungs. Pulmonary compliance is calculated as the ratio of the change in lung volume to the change in transpulmonary pressure—that is,
where
Compliance denotes distensibility, the ease of stretch or inflation. The inverse of compliance (i.e., elastance) refers to the stiffness or the tendency to resist distortion and to return to the original configuration when the distorting force is removed.
In practice, pulmonary compliance is determined by relating the changes in transpulmonary pressures to the changes in lung volume during interruptions in the course of an expiration after a maximal inspiration (i.e., starting from TLC).
The pressure–volume characteristics of the lung are nonlinear. As lung volume increases, the elastic elements approach their limits of distensibility, and a given change in transpulmonary pressure produces smaller and smaller increases in lung volume.6,7 Thus, the compliance of the lung is least at high lung volumes and greatest as RV is approached (Fig. 10-2). Elastic recoil forces favoring collapse of the lung can be demonstrated throughout the range of the vital capacity, even at low lung volumes approaching the RV. If the opposing forces of the chest wall on the lungs are eliminated – for instance, by removing the lungs from the thorax or by opening the chest – the lung collapses to a near-airless state. A minimal volume of air does remain in the lungs because of closure of small airways resulting in the trapping of air in more distal airspaces.
Figure 10-2 Pressure–volume curve of the lung. The static elastic recoil pressure of the lung is approximately 5 cmH2O at FRC and 30 cmH2O at TLC. The compliance of the lung (ΔV/ΔP) is greater at low lung volumes than at high lung volumes.
If static measurements of transpulmonary pressure are made during lung inflation rather than deflation, the pressure–volume curve has a different configuration (Fig. 10-3). This indicates that the elastic recoil of the lung depends not only on the lung volume at which the determination is made but also on the “volume history” of the lung.8
Figure 10-3 Pressure–volume curves of the lung during inspiration and expiration.
HYSTERESIS
Differences in the pathways of the static pressure–volume curve during inspiration (when force is applied) and expiration (when force is withdrawn) are designated as hysteresis, which is a property of all elastic structures. In the lungs, it is due to the surface forces and the properties of the surface material lining the alveolar walls and also to the elastic properties of the tissues. The tissues of the lung are also subject to stress adaptation whereby over time, the pressure required to maintain a given lung volume will decline.9 An additional factor relates to the closure of small airways at low lung volumes. Once these airways close, the lung units that they serve will not expand during inspiration until a critical opening pressure has been exceeded; only then will the closed units inflate. Recruitment of additional lung units as increasing transpulmonary pressure expands the lungs from low lung volume contributes to the hysteresis of the pressure–volume curve.
The elastic behavior of the lung depends on two factors: the physical properties of the lung tissue, per se, and the surface tension of the film lining of the alveolar walls.
SURFACE FORCES
The interior surfaces of the alveoli are lined by a thin liquid layer of osmophilic material. The surface tension at the air–liquid interface of the alveoli, in addition to the elastic properties of the parenchyma, contributes importantly to the elastic recoil of the lungs and acts to decrease lung compliance.10 The cohesive forces between the molecules of the liquid lining of the alveoli are stronger than those between the film and alveolar gas, thereby causing the film to shrink to its smallest surface area. The behavior of this surface film has been examined in experimental animals by comparison of pressure–volume relationships of air-filled lungs with those of saline-filled lungs; saline eliminates the liquid–air interface without affecting elastic properties of the tissue. A lung distended with saline requires a lower transpulmonary pressure to maintain a given lung volume than a lung that is inflated with air.11 Also, hysteresis is less in the saline-filled lung. The greater hysteresis in the air-filled lung is explained by the surface tension of the film lining the alveoli, which is higher during inflation as the film expands than it is during deflation as the film is compressed (Fig. 10-4).
Figure 10-4 Comparison of pressure–volume relationships of air-and saline-filled excised lungs. Arrows directed upward indicate inflation; those directed downward indicate deflation. Since saline eliminates surface forces at the liquid–air interface without affecting tissue elasticity, the difference in pressure between the two curves, at any lung volume, is that required to overcome surface forces. To maintain a small lung volume, a large proportion of the pressure is used to overcome surface forces. In contrast, at high lung volumes a greater fraction of the pressure is used to overcome tissue elasticity.
By considering the alveolus to be a sphere, Laplace’s law can be applied. Laplace’s law states that the pressure inside a spherical structure—for example, the alveolus—is directly proportional to the tension in the wall and inversely proportional to the radius of curvature:
where
Abolition of the liquid–air interface by the instillation of saline into the alveolar spaces eliminates surface forces, thereby reducing the transpulmonary pressure required to maintain a given lung volume.
The surface film lining the alveoli of the lung is termed surfactant.12 The superficial layer of the film facing the alveolar air is made up of surface-active phospholipids, notably dipalmitoyl lecithin. The deeper layer termed the hypophase consists of surface-active phospholipids linked to protein. Surfactant is generated by type II alveolar cells and undergoes a continuous cycle of formation, removal, and replenishment.13
Surfactant serves several important functions. The surface tension of surfactant is inherently low and decreases even further at low lung volumes when the surface area of the film is reduced. The minimization of surface forces, particularly at low lung volumes, minimizes the adherence of the walls of distal airways that tend to close at low lung volumes and increases the compliance of the lung and decreases the work required to inflate the lungs during the next breath. The automatic adjustment of surface tension as lung volume changes also promotes stability of alveoli at low lung volumes; if the surface tension were to remain constant instead of changing with lung volume, the transpulmonary pressure required to keep an alveolus open would increase as the radius of curvature diminished with decreasing lung volume. Therefore, small alveoli would empty into the larger ones with which they communicate, and atelectasis would be a regular occurrence (Fig. 10-5). Surfactant dysfunction as occurs with acute lung injury results in marked increases in surface tension causing stiffening and instability of alveoli and leads to alveolar collapse.
Figure 10-5 The effects of surfactant in maintaining alveolar stability. A. Surfactant lowers the tension of the alveolar walls at low lung volumes. Consequently, the transpulmonary pressure (P) of large and small communicating airspaces is the same. r1 < r2, T1 < T2, P1 ← P2. B. Without surfactant, the surface tension remains constant as lung volume changes, and the recoil pressure of small airspaces exceeds that of larger ones. As a result, small alveoli tend to empty into larger ones. r1 < r2, T1 = T2, P1 > P2.
INTERDEPENDENCE AND COLLATERAL VENTILATION
The low surface tension of surfactant is not the most important determinant of alveolar stability. In reality, the alveoli form a froth rather than individual bubbles.14 The walls of each alveolus are shared in common with those of adjacent alveoli so that contiguous airspaces attached by their connective tissue framework are tethered to one another and are not free to move independently. The tendency of any one alveolus to collapse is opposed by the traction exerted by the surrounding alveoli. This mechanical interdependence of adjacent airspaces resists the collapse of individual alveoli and serves as a stabilizing influence and ensures uniform inflation.15 Even when a distal airway is completely obstructed, the alveoli served by the airway can still be ventilated through collateral channels between alveoli (pores of Kohn) and from bronchioles to alveoli (canals of Lambert). This collateral ventilation also prevents alveolar collapse and enhances the uniformity of ventilation, particularly in patients with lung disease.16
PHYSICAL PROPERTIES OF LUNG TISSUE
A number of different tissue components contribute to lung elasticity. The pleura, the intralobular septa, peripheral airway smooth muscle tone, and pulmonary vasomotor tone, as well as the tissues of the alveolar walls, play a role in shaping lung elastic recoil.
The major connective-tissue elements of the alveolar walls are the collagen and elastin fibers.17 Elastin fibers in the alveolar walls and surrounding the bronchioles and pulmonary capillaries have a low tensile strength but can be stretched to over twice their resting length. Elastin fibers are thought to bear most of the stress in the lung at low volumes. Collagen fibers have high tensile strength but are poorly extensible and probably act to limit expansion at high lung volumes.18 Like a stretched nylon stocking, expansion of the lungs appears to entail an unfolding and geometric rearrangement of the fibers and only slight elongation of individual fibers.
As a result of alterations in the elastin and collagen fibers in the lung, the distensibility of the lungs (measured as compliance) increases with age.7 This is part of the normal aging process. Pulmonary compliance is also increased by the destruction of alveolar walls and the enlargement of alveolar spaces that characterize pulmonary emphysema. In contrast, the distensibility of the lungs is reduced by pulmonary fibrosis, which stiffens its interstitial tissues.6
ELASTIC PROPERTIES OF THE THORAX
The elastic recoil of the chest wall is such that if it were unopposed by the lungs, the chest would enlarge to approximately 70% of TLC. This position represents its equilibrium or resting position.19 In this position (when the respiratory muscles are completely relaxed), the pressure difference across the chest wall – that is, the difference between pleural pressure and the pressure at the surface of the chest – is zero. If the chest were forced to enlarge beyond its equilibrium position by an increasingly positive pleural pressure or by the application of subatmospheric pressure at the body surface, it would, like the lung, recoil inward, resisting expansion and favoring return to its equilibrium position. Conversely, at volumes less than 70% of TLC, the recoil of the chest is opposite that of the lung and is directed outward (Fig. 10-6).20 The chest wall can also be represented as a two-compartment system consisting of the rib cage and the abdomen, and volume changes can be partitioned between the two compartments.21 Changing from the upright to the supine position at a constant overall lung volume produces a shift in volume from the abdominal to the rib cage compartment. The compliance of the rib cage is similar in the supine and upright positions, but the compliance of the abdominal compartment – particularly at high volumes – is greater in the supine position.22
Figure 10-6 Pressure–volume relationships of the isolated chest wall. The direction of the recoil forces across the chest wall is represented by the arrows.
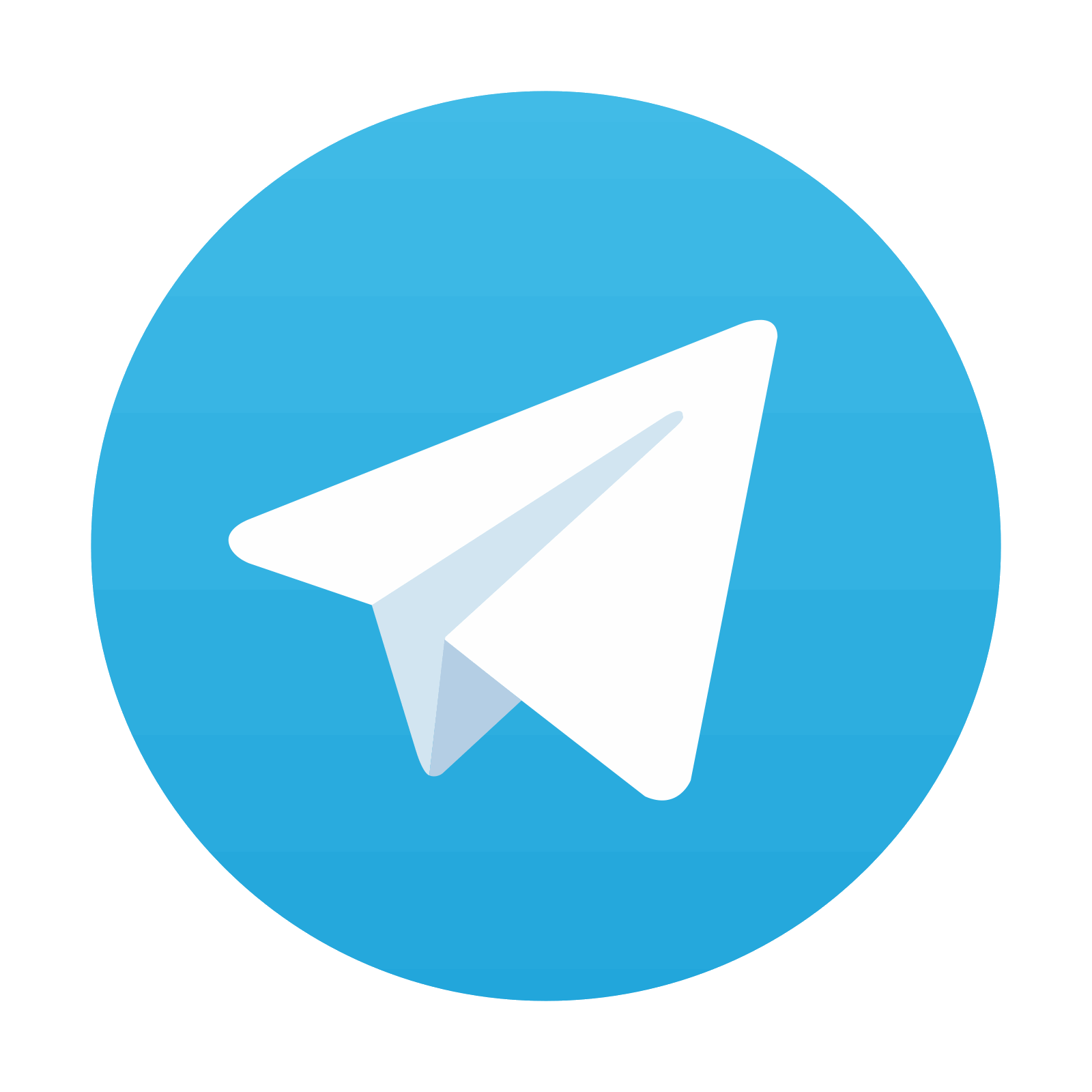
Stay updated, free articles. Join our Telegram channel
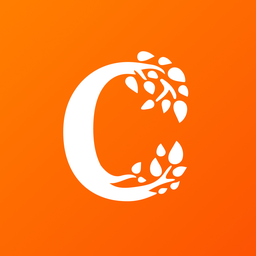
Full access? Get Clinical Tree
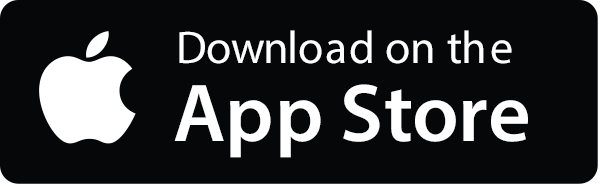
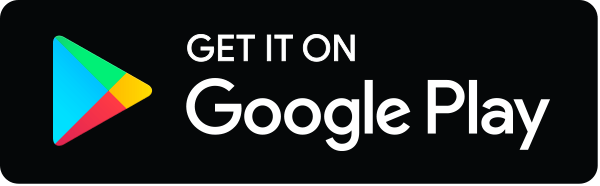