Introduction
Pulmonary edema—defined as excessive extravascular water in the lungs—is a common and serious clinical problem. Pulmonary edema can be life-threatening, but effective therapy is available to rescue patients from the deleterious consequences of disturbed lung fluid balance, which usually can be identified and, in many instances, corrected. Because rational and effective therapy depends on understanding basic principles of normal and abnormal liquid, solute, and protein transport in the lungs, this chapter begins with a brief overview of the major factors that govern fluid and protein filtration in healthy lungs before focusing on the pathophysiology of pulmonary edema. Next, the chapter discusses diagnosis, treatment, and resolution of pulmonary edema. Chapters 6 and 9 also provide additional information about the regulation of fluid balance in the lungs, and Chapter 100 includes details about the onset and management of acute lung injury and acute respiratory distress syndrome, as currently defined and subsequently discussed.
Pathophysiology of Pulmonary Edema
Pulmonary edema results when fluid is filtered into the lungs faster than it can be removed from them. Accumulation of fluid has serious consequences on lung function because gas exchange is greatly impaired in fluid-filled alveoli. Lung structure relevant to the forces governing fluid and protein movement in healthy lungs and lungs with pulmonary edema has been the subject of classic and more recent reviews.
There is always a net outward flux of fluid and protein crossing from the vascular space into the interstitium in the lungs, first, because the prevailing driving forces normally cause filtration out of the bloodstream and, second, because the microvascular endothelium is a permeable barrier that varies in its leakiness. Lung lymph flow, which represents the flow of fluid leaking across the microvascular barrier, normally is less than 0.01% of total lung blood flow. The term microvascular bed (or barrier ), is used throughout this chapter to refer to sites of fluid exchange. In addition to the vast interconnecting network of capillaries embedded in the alveolar walls, fluid is exchanged across capillaries in the interstitium at alveolar wall junctions (corner vessels) and across small interstitial arteries and veins.
The essential factors that govern fluid exchange in the lungs are expressed in the Starling equation for the microvascular barrier:
Jv = LpS [ ( Pc − Pi ) − σ d ( π c − π i ) ]
The microvascular hydrostatic pressure is the principal force that causes fluid filtration in the lungs. If blood was not flowing through the lungs, the opposing hydrostatic and osmotic forces on either side of the microvascular barrier would be equal, their sum would be zero, and there would be no filtration. The pumping action of the heart causes blood to flow through the lungs and generates the microvascular hydrostatic pressure that establishes the steady-state values of the other driving pressures that cause filtration of fluid.
According to the Starling equation, the difference between the prevailing transmural hydrostatic pressures (Pc − Pi) and the colloid osmotic pressures (πc − πi) provides the “driving force” for fluid filtration. The actual amount of filtrate that forms at any given driving force is determined by the integrity of the barrier to filtration, which is reflected in the conductivity (Lp) and reflection (σd) coefficients. The equation predicts the development of two fundamentally different kinds of pulmonary edema: (1) increased pressure pulmonary edema— when the net result of the driving forces increases, fluid filtration is forced across the barrier at a rate that exceeds removal by lymphatic drainage, and (2) increased permeability pulmonary edema— when the normal barriers to fluid filtration are damaged, typically by some form of injury, conductance of liquid and protein in the lungs is allowed to increase. A third type of pulmonary edema is caused by impaired lymphatic drainage of filtered fluid, but this has less clinical relevance than the other two types. The lymphatic drainage of the lungs provides a vital means for removing filtered fluid and proteins from the perimicrovascular interstitial space, as discussed later.
Because the healthy microvascular barrier is permeable, the alveolar barrier must serve as the principal protection against the accumulation of pulmonary edema. Fluid and protein do not normally move into alveoli because the alveolar epithelial barrier has a low permeability even to small molecules (similar to cell membrane permeability); in addition, any fluid that is filtered is continuously being pumped back into the interstitium by alveolar epithelial cells, drained away from the alveolar walls through the interstitium, and removed by lymphatic vessels and the lung microcirculation.
The several factors ( Table 62-1 ) that normally protect the lungs against edema have been called safety factors. Under normal conditions, the lymphatic system pumps filtered fluid and protein out of the lungs as rapidly as they are formed, even when filtration of fluid and protein from the bloodstream into the interstitium is increased. Increases in fluid and protein filtration across the microvascular barrier also can be drained away from the alveolar walls down the prevailing pressure gradient into the loose peribronchovascular connective tissue or can be resorbed directly into blood vessels. The lung lymphatics can increase their pumping capacity manifold, particularly when the microvascular wall has been injured.
|
When the usual driving forces are upset by higher hydrostatic pressure, the increase in filtration of water across the microvascular barrier is much larger than that in protein flux because the microvascular barrier has a low protein conductance. This results in dilution (“wash-down”) of interstitial protein concentration and, thereby, an increase in the balance of the protein osmotic pressure opposing the higher hydrostatic pressure (because plasma protein concentration remains high). Furthermore, the interstitial gel also becomes hydrated and the exclusion volume for protein decreases, either because of swelling or because its composition changes as hyaluronan is washed out from the interstitium, reducing the concentration of protein by expanding the available volume.
The protein osmotic pressure safety factors work only when the microvascular barrier is normal—as in increased pressure edema. In contrast, if the endothelial barrier is injured and its functional integrity is compromised—as in increased permeability edema—barrier conductance increases and the osmotic reflection coefficient decreases, making this safety factor much less effective or even completely ineffective. The compliance of the interstitial space also protects the lungs against edema. Increases in interstitial volume result in only small elevations of interstitial pressure until the interstitial volume is large. This maintains the hydrostatic driving pressure across the alveolar barrier suitably low. When interstitial pressure within the lungs rises to greater than pleural pressure, fluid flows across the visceral pleura into the pleural space, where its effects on lung function are relatively minor. Pleural fluid is drained by lymphatics in the parietal pleural and, even when pleural liquid accumulates, does not flow back from the pleural space into the lungs. Fluid that does accumulate in the alveoli is pumped out by active ion transport. There are several mechanisms that can up-regulate the rate of alveolar fluid clearance (see Chapter 9 ).
In summary, pulmonary edema results from increases in either driving pressures (increased pressure edema) or barrier conductance (increased permeability edema) or both combined. What distinguishes between the two types is barrier permeability, which is normal in increased pressure edema but leaky in increased permeability edema. Fluid flow into the lungs is driven across the barrier in both types of edema according to the prevailing pressures.
Increased Pressure Edema
Increased pressure pulmonary edema ( Fig. 62-1 ) is caused by an increase in the net sum of driving forces for fluid filtration into the lungs. The essential feature of this edema is that the barriers to fluid and protein flow into the lungs are functionally intact. Increased pressure edema is often called cardiogenic, high-pressure, or hydrostatic pulmonary edema .

Pathophysiology
The flow of fluid and protein into the lungs increases when the sum of driving pressures is elevated. If the rate of fluid accumulation exceeds the rate at which it can be removed, increased pressure edema results. Because the barriers limiting fluid and protein flow into the lungs are intact, the lungs are protected against edema by the prevailing (normal) safety factors. Especially important is the ability to protect against increases in the principal driving pressure, lung microvascular hydrostatic pressure. Because of the low protein conductance of the microvascular barrier, fluid flow increases much more than protein flow when microvascular hydrostatic pressure rises. Interstitial protein concentration is diluted both by this higher fluid flow relative to protein flow and by a diminished exclusion volume for proteins as the interstitial gel is hydrated and swells. Lower interstitial protein concentration, owing to washout of interstitial proteins, results in lower perimicrovascular protein osmotic pressure and thereby a greater protein osmotic pressure difference across the barrier, which opposes any rise in hydrostatic pressure. In experimental animals, slightly less than 50% of an increase in hydrostatic pressure is offset by the increase in osmotic pressure difference. Increased pressure edema may be gradual in onset and progression because any elevation in microvascular hydrostatic pressure is attenuated by a rise in protein osmotic pressure difference across the microvascular barrier, owing to a decline in interstitial protein osmotic pressure.
The consequences of increased pressure edema on lung mechanics and gas exchange ( Table 62-2 ) depend on how much edema accumulates. Deliberate dehydration of healthy subjects increases lung volumes and improves tests of ventilatory function. Early in the development of pulmonary edema, increases in hydrostatic pressure result in enlargement of the intrapulmonary blood volume, as vessels, including capillaries, are both recruited and distended, which causes the diffusing capacity of the lung (D l CO ) to increase above normal; similarly, arterial oxygen pressure (P o 2 ) may rise because ventilated units are better perfused when vascular pressures increase. The small reversible changes in airflow resistance and dynamic compliance, unaffected by vagotomy, in congested lungs appear to be due to reflex bronchoconstriction responses, but only when baseline bronchial tone is normal.
VASCULAR CONGESTION |
Increased diffusing capacity |
Increased arterial P o 2 |
Decreased compliance |
Bronchoconstriction |
INTERSTITIAL EDEMA |
Increased closing volume |
Decreased maximal expiratory flow |
Increased ventilation-perfusion mismatching |
Decreased arterial P o 2 |
ALVEOLAR FLOODING |
Increased closing volume (air trapping) |
Increased vascular resistance |
Decreased lung volume (especially vital and inspiratory capacities) |
Decreased compliance |
Decreased diffusing capacity |
Right-to-left shunting of blood (severely compromised gas exchange) |
When interstitial edema is present, closing volume may be increased and maximum expiratory airflows may be decreased. These changes were originally thought to be due to a decrease in caliber of small airways caused by compression by rising volume and pressure in the peribronchovascular connective tissue spaces. This effect would have to be in airways larger than bronchioles, because bronchioles and smaller airways do not have loose connective tissue sheaths and their diameter is a function of lung volume, not transpulmonary pressure. Arterial P o 2 often falls as a result of ventilation-perfusion mismatching, but gas exchange is not seriously compromised until the alveoli are flooded.
With alveolar flooding, lung volumes are diminished. This is most marked in measurements of vital capacity, with inspiratory capacity affected more than expiratory capacity. Airways may close at higher than normal distending pressures, resulting in trapping of larger volumes of gas in the lungs. Lung compliance is reduced when alveolar edema is present because lung volume decreases. Gas exchange becomes severely compromised when flooded alveoli remain perfused, causing right-to-left shunts , and there is an increase in wasted ventilation (ventilation of units in which there is decreased or absent perfusion).
Light and electron microscopic examination of human and animal lung tissue in increased pressure edema shows alveolar edema and hemorrhage; thickened interstitial compartments (especially large peribronchovascular fluid cuffs) with separated, dispersed collagen fibrils; and increased capillary surface area and volume. More intercellular vesicles can be seen, but otherwise there are no detectable changes in the ultrastructure of the vascular endothelium, and the gap widths at intracellular junctions are not different from those in normal lungs. Long-standing pulmonary edema (e.g., in patients with chronic mitral stenosis) can be associated with basement membrane thickening and increased distance between the alveoli and the capillaries; increases in fibroblasts, histiocytes, and bulky strands of collagenous fibers can be seen in the interstitium. Dogs with pacing-induced chronic congestive heart failure developed a significant increase in the threshold for high-vascular-pressure edema formation—about a 50% reduction in the amount of water and protein cleared across the lung microvascular endothelial barrier at high pulmonary vascular pressures—compared with control animals. Morphometric analysis of the alveolar-capillary barrier showed that endothelial, interstitial, and epithelial thicknesses were all increased compared with controls, indicating that remodeling may confer an increase in the resistance to development of high-pressure–induced alveolar edema. Alveolar type II cells may be more numerous than in normal lungs, and alveolar macrophages proliferate. Sites of chronic severe increased pressure edema may also become organized and fibrotic, may calcify, and may even result in bone formation.
Mechanisms
By far the most common cause of increased pressure edema is elevated lung microvascular hydrostatic pressure. The influence of driving pressures would be greater than usual if either perimicrovascular hydrostatic pressure or protein osmotic pressure difference across the microvascular barrier was decreased. At the alveolar barrier, an increase in interstitial hydrostatic pressure, a decrease in alveolar hydrostatic pressure, or a decrease in osmotic pressure difference across the barrier could result in a greater sum of driving pressures. The possibilities are listed in Table 62-3 .
INCREASED LUNG MICROVASCULAR HYDROSTATIC PRESSURE |
Left ventricular dysfunction |
Mechanical obstruction of left atrial outflow |
Volume overload |
Pulmonary venous hypertension |
Overperfusion |
Increased lymphatic outflow pressure |
Decreased perimicrovascular hydrostatic pressure |
Inspiratory airway obstruction |
Increased alveolar surface tension |
Increased Microvascular Hydrostatic Pressure.
Congestive heart failure is the most common cause of increased pressure edema. That is why increased pressure edema is often called “cardiogenic,” even though the heart is not always primarily involved. Elevated pressures in the pulmonary microvasculature are usually due to left-sided heart failure, with elevated left atrial pressures transmitted retrograde into the pulmonary circulation. Common causes are left ventricular dysfunction (e.g., caused by acute myocardial infarction, severe coronary insufficiency, tachyarrhythmias, bradyarrhythmias, cardiomyopathies, constrictive pericarditis, aortic stenosis or regurgitation, mitral regurgitation, coarctation of the aorta, rupture of chordae tendineae or intraventricular septum, systemic hypertension) or mechanical obstruction of the left atrial outflow tract (e.g., mitral stenosis, left atrial myxoma). Left atrial and pulmonary microvascular pressures can also be elevated by severe fluid volume overloading in a patient with a normal or diseased heart.
An unusual cause of increased microvascular hydrostatic pressure is pulmonary venous hypertension in the absence of left ventricular or atrial disease, which can arise if the pulmonary veins are contracted (e.g., by possible muscular sphincters), compressed, or obstructed (e.g., because of veno-occlusive disease or mediastinal fibrosis). Bronchial venous hypertension, in contrast, does not appear to significantly increase fluid filtration in the lungs.
Increases in fluid filtration also can be associated with increases in vascular pressure proximal to the filtration sites in the lungs. For example, pulmonary hypertension, combined with depressed left ventricular function, has been implicated in the pathogenesis of cocaine-induced pulmonary edema. Whether such increases lead to pulmonary edema depends on what happens to microvascular pressure. If high right-sided pressures are caused by increased resistance proximal to the main site of filtration in the lungs—as found in hypoxic pulmonary vasoconstriction of small arterial vessels, primary pulmonary hypertension, and pulmonary artery or valvular stenosis—pulmonary edema does not develop. Conversely, if the lung vascular bed is only partially constricted or obstructed, or if the vascular surface area is greatly decreased (e.g., by lung resection), higher flow in perfused vessels can lead to increased pressure edema, because microvascular pressures at the fluid exchange site are elevated in the overperfused lung. For example, pulmonary edema resulted in about 15% of patients after pneumonectomy and seemed to be exacerbated by administration of fresh frozen plasma, in part because intravascular volume is presumably increased by such transfusions. Any increase in blood flow through the lungs increases the pulmonary microvascular pressure at the fluid exchange sites even when pulmonary venous pressure remains constant.
The mechanism of an uncommon cause of pulmonary edema, high-altitude pulmonary edema, which is also discussed in Chapter 77 , may also be related, in part, to elevated pulmonary vascular pressures. As noted earlier, overperfusion of a restricted pulmonary vascular bed, even in the absence of hypoxia, causes increased pressure edema, not increased permeability edema. This can explain why some cases of high-altitude pulmonary edema are correctly classified as increased pressure pulmonary edema. Evidence from climbers studied at high altitude suggests that high intravascular pressures cause physical damage to vascular walls (so-called stress failure). In experimental animals, stress failure has been demonstrated after extreme, but sometimes transient, increases in pulmonary vascular pressures. Such structural failures need not happen in large numbers to explain an increase in permeability edema, because edema would form readily and in a quantity driven by the prevailing elevated vascular pressure. The suggestion has been made that high-altitude pulmonary edema results from the stress failure of overdistended, relatively thin-walled pulmonary arteries rather than from microvascular rupture, which might help explain why the prevailing vasoconstriction does not seem to offer much protection to downstream vessels, why there are no reports of gradual progression through an indolent prodrome of increased pressure edema before stress failure results, and why high-altitude pulmonary edema is first detected radiographically in the central lung fields surrounding large vessels rather than in the lung bases and periphery.
Alternative mechanisms for the increased permeability in high-altitude pulmonary edema have been proposed. However, inflammatory responses in high-altitude pulmonary edema may be a consequence rather than a cause of the edema. The rapid reversibility of high-altitude pulmonary edema with descent to lower elevation, oxygen therapy, or pharmacologic reduction of pulmonary vascular pressure is not characteristic of increased permeability pulmonary edema with coexisting inflammation.
Neurogenic pulmonary edema also may be related in part to elevated pulmonary vascular pressures ( Fig. 62-2 ). Measurements of edema fluid protein concentration relative to plasma protein concentration in 12 patients with neurogenic pulmonary edema have been reported. Seven of the patients had ratios typical of increased pressure pulmonary edema, and the other five had ratios consistent either with increased permeability pulmonary edema or with late sampling during the resolution phase of increased pressure pulmonary edema, because edema fluid protein concentration rises as fluid is reabsorbed from the alveoli at a faster rate than protein.

Decreased Perimicrovascular Hydrostatic Pressure.
The sum of driving pressures would increase if perimicrovascular hydrostatic pressure was greatly diminished, thereby resulting in an increase in fluid and protein filtration at the microvascular barrier in the lungs. Pulmonary edema has been described in circumstances in which this might happen. The best clinical example may be postobstructive pulmonary edema as a consequence of upper airway obstruction or its release, which can be caused, for example, by laryngospasm, endotracheal tube obstruction, foreign body aspiration, epiglottitis, croup, severe acute asthma, airway compression by tumors, strangulation, or hanging. High negative intrathoracic pressures generated by inspiratory attempts against the occluded airway are transmitted to the interstitium, promoting fluid movement into the interstitium. Mechanical effects on the cardiovascular system likely contribute to this kind of edema. High negative intrathoracic pressure causes increases in cardiac preload and afterload and in pulmonary blood flow, all of which increase the microvascular pressure that drives fluid out into the interstitium. In three patients with upper airway obstruction and pulmonary edema, all had low edema fluid protein concentration relative to plasma protein concentration (ratios of 0.44, 0.31, and 0.52), indicating increased pressure pulmonary edema.
Aspiration of air or fluid from the pleural space with consequent reexpansion of a collapsed lung could result in a decrease in perimicrovascular hydrostatic pressure as the lung expands to fill the thorax. So-called reexpansion pulmonary edema has been reported both in experimental animals and in patients after lung reexpansion ( Fig. 62-3 ), but the high edema fluid protein concentration measured in three patients indicated that reexpansion may result in an increased permeability edema rather than an increased pressure edema. However, another study of protein concentration in pulmonary edema fluid from patients with reexpansion edema indicates that hydrostatic mechanisms predominate. The increased permeability hypothesis was supported in studies of rabbits with experimental reexpansion edema. Reperfusion injury is one of the causes of the pulmonary edema seen in transplanted lungs and appears to be predominantly an increased permeability edema.

If high alveolar surface tension was transmitted to the interstitium, perimicrovascular hydrostatic pressure would also be lowered, thereby increasing filtration across the microvascular barrier. Such an effect has been suggested by experimental findings in dog lungs. The effect of alveolar surface tension on lung fluid balance is discussed later.
Decreased Transmural Protein Osmotic Pressure Difference.
The sum of driving pressures would be increased if the protein osmotic pressure difference opposing the hydrostatic pressure difference across the microvascular barrier was decreased, either by lowering plasma protein concentration or by raising interstitial protein concentration, resulting in an increase in the sum of driving pressures for fluid and protein flow into the lungs. This theoretical mechanism of increased pressure edema has been the subject of study in experimental animals, with contradictory results. When plasma protein osmotic pressure is low, the ability of the osmotic pressure gradient to widen in response to increased hydrostatic pressure is diminished, and edema accumulates at hydrostatic driving pressures lower than those needed to cause edema when protein concentration is normal.
Alveolar Barrier Function.
If interstitial hydrostatic pressure was raised or if alveolar hydrostatic pressure or the osmotic pressure difference across the alveolar barrier was lowered, driving pressure for fluid and protein flow across the alveolar barrier would be elevated, resulting in increased pressure edema. Interstitial hydrostatic pressure rises as interstitial edema accumulates in the lungs. Increased interstitial hydrostatic pressure would raise the sum of driving pressures across the alveolar barrier and could drive edema formation across the alveolar or airway epithelium.
Greater pressure filtration across the alveolar barrier would also result from an increase in the sum of driving pressures if alveolar hydrostatic pressure was lowered. This is complicated by the interrelation between alveolar and interstitial hydrostatic pressures. A drop in alveolar hydrostatic pressure, which increases the sum of driving pressures across the alveolar barrier, also results in a lowering of interstitial hydrostatic pressure, which decreases the sum of driving pressures across the alveolar barrier but increases the sum of driving pressures across the microvascular barrier. Administration of a detergent aerosol resulted in loss of surfactant activity, higher alveolar surface tension, lower static compliance, atelectasis, and pulmonary edema; low protein concentration in alveolar edema fluid and left-hilar afferent lymph relative to plasma protein concentration indicated that this was an increased pressure type of pulmonary edema. Because increased pressure edema can impair surface activity of dog lung extracts and isolated rabbit lungs, it is possible that changes in alveolar surface tension may accelerate edema formation. However, the notion that changes in alveolar surface tension in edema lead to a self-perpetuating vicious circle of edema formation is not borne out clinically.
The only kind of clinical pulmonary edema caused by transmural osmotic pressure differences is near-drowning. Seawater is three times more hyperosmotic (1000 mOsm) than plasma, so the volume of fluid in the air spaces after saltwater aspiration increases threefold to reach osmotic equilibrium, thus markedly increasing the alveolar edema already present owing to the volume of aspirated seawater itself in the alveoli. Osmotic equilibrium is reached in minutes as water is drawn from neighboring blood vessels into the alveoli by osmotic pressure. Alveolar barrier function is not significantly compromised (unless perhaps the patient aspirates gastric contents or the seawater is contaminated or rich in particulate matter), and the alveolar edema is cleared rapidly (50% to 60% of excess alveolar fluid is cleared in 4 hours). Freshwater near-drowning proceeds in the opposite fashion: osmotic equilibrium is reached rapidly by flow of water out of the alveoli into the interstitium and bloodstream. Rapid water flux and hypotonicity can cause severe hemodilution, with hemolysis and fibrinolysis, as well as severe distortion of pulmonary ultrastructure, including damage to type I and type II cells, endothelial cell swelling, basement membrane detachment, and cell disruption. Both the alveolar epithelium and microvascular endothelium can thus be injured by the hypotonic fluid, leading to increased permeability pulmonary edema rather than a normal barrier type of pulmonary edema.
Increased Permeability Edema
Increased permeability pulmonary edema ( Fig. 62-4 ) is caused by an increase in liquid and protein conductance across the barriers in the lungs. The essential feature of this edema is that the integrity of the barriers to fluid and protein flow into the lung interstitium and alveoli is altered from lung parenchymal damage. Increased permeability edema is sometimes called noncardiogenic pulmonary edema, and the resulting clinical syndromes in humans—when explicitly defined (see later)—are referred to as acute lung injury (ALI) or, when severe, the acute respiratory distress syndrome (ARDS).

Pathophysiology
If the rate of fluid and protein accumulation from lung endothelial and epithelial barrier injury exceeds the rate at which it can be removed, increased permeability edema results. Because the barriers limiting fluid and protein flow into the lungs do not function normally when the lungs are injured, the lungs are not protected against edema by the usual safety factors. Although increases in fluid and protein filtration across the barriers are removed by lymphatics and drained away from the alveolar walls as in increased pressure edema, much more fluid and protein are filtered at any given sum of driving pressures because the barriers to their flow are much less restrictive than normal. Edema formation in injured lungs becomes extremely sensitive to driving pressures. Driving pressures are often increased when the lungs are injured because of the vasoconstrictive effects of inflammatory mediators such as thromboxanes, which may shift the main site of resistance to postcapillary venules, thus increasing hydrostatic pressure at the microvascular fluid exchange sites, or because of effects on the heart as well as on the circulation. For example, elevated left atrial pressure, pulmonary venoconstriction, and an increase in cardiac output in sepsis can increase hydrostatic pressure at the microvascular fluid exchange sites.
Because the endothelial-epithelial barrier becomes leaky, protective protein osmotic pressure differences are lost across them, driving pressure is unopposed by protein osmotic pressure, and even normal hydrostatic pressure results in significant fluid and protein extravasation into interstitial and alveolar spaces. The ability of the lymphatics to pump the excess filtrate away is increased when the lungs are injured. Maximal lung lymph flow increases more when the microvascular wall has been injured than when hydrostatic pressure alone is increased, but even this augmented lymphatic pumping capability is taxed at low driving pressures. If the epithelial barrier is injured, edema may accumulate readily in the alveoli, because most of the resistance to fluid and protein flow into the alveoli resides in the epithelial barrier. Increased permeability edema is often rapid in onset and progression because injured barriers offer much less resistance to flow and because hydrostatic driving pressure is unopposed by increases in osmotic pressure difference. Clinically, patients with increased permeability edema usually have a low intravascular hydrostatic pressure, commonly measured as a low or normal pulmonary capillary wedge pressure. In some cases, this reflects the low intravascular pressures associated with the underlying disease process (e.g., sepsis).
The consequences of increased permeability edema on lung mechanics and gas exchange depend on how much edema accumulates and how severe the causative lung injury is. As with increased pressure edema, the major effects on pulmonary mechanics follow alveolar flooding. In experimental lung injury, functional residual capacity decreases as a consequence of alveolar flooding, and this loss of ventilated units accounted for virtually all the observed decrease in static lung compliance. Computed tomography (CT) has provided new insights into structure-function relationships in human ALI. In its early stage, when alveolar edema predominates, the lungs are characterized by a homogeneous alteration of vascular permeability, and edema accumulates evenly in all lung regions with a nongravitational distribution. Increased lung weight due to edema causes collapse of lung regions along the vertical axis through the transmission of hydrostatic forces (compression atelectasis, caused by the weight of edema). Thus lung volume is lost mainly in the dependent lung, where the superimposed weight from above is greatest.
Measurements of pulmonary mechanics in mechanically ventilated patients with diffuse parenchymal lung damage showed decreased static lung compliance as a consequence of loss of ventilated lung. In addition, airflow resistance was increased as a result of decreased lung volume. Bronchospasm may add to the increase in airflow resistance and can be substantially reversed by bronchodilator inhalation. Chest wall compliance was reduced, probably because of alterations of intrinsic mechanical properties of the chest wall by abdominal distention, chest wall edema, and pleural effusion. Different respiratory mechanical abnormalities and responses to positive end-expiratory pressure (PEEP) during mechanical ventilation were reported in patients with severe increased permeability edema originating from pulmonary disease (pneumonia with consolidation) or from extrapulmonary disease (associated with pulmonary edema and alveolar collapse).
Although the effects of surface forces on decreased lung compliance in patients with diffuse lung damage were thought to be small, results of experiments in isolated rabbit lungs indicated that increased permeability edema may result in more severe mechanical changes than equivalent degrees of increased pressure edema. Surfactant is thromboplastic, and coagulation may compound surfactant depletion when plasma proteins enter the air spaces. The injured lung may release substances that interfere with the normal low surface tension in the alveoli, and activated polymorphonuclear leukocytes (PMNs) impair surfactant function in vitro and degrade the major surfactant apoproteins by proteolysis and oxidant-radical–mediated mechanisms. Human lung surfactant obtained by bronchoalveolar lavage (BAL) of patients at risk for diffuse lung damage and patients with established injury is abnormal in chemical composition and functional activity. Abnormalities also could be caused by interactions between surfactant and edema proteins, because plasma proteins (especially fibrin monomers but also fibrinogen and albumin) interfere with surfactant function. Proteinaceous edema fluid has been associated with surfactant inhibition in various experimental models. The role of surfactant in the development and treatment of diffuse parenchymal lung damage and the potential role for surfactant therapy are discussed in more detail later in this chapter.
Gas exchange is often severely compromised in increased permeability edema, owing both to intrapulmonary shunting of blood and to ventilation-perfusion inequalities. Patients with early lung injury typically have a marked increase in their pulmonary dead-space fraction, indicating that many ventilated lung units are not well perfused, although intrapulmonary shunting may also contribute to the elevated dead space ; this finding explains why minute ventilation rises to twice normal (12–16 L/min) at the onset of severe increased permeability edema. An elevated pulmonary dead-space fraction also has been reported in pediatric patients with widespread lung injury, the mechanism for which may in part be explained by an increase in procoagulant and antifibrinolytic pathways in parenchymal damage.
The physiologic abnormalities associated with increased permeability edema are dominated by early alveolar flooding and depend on the severity and the duration of injury as well as its cause. Manifestations may resolve or worsen, but typically evolve in three pathologic patterns: exudative, proliferative, and fibrotic, usually in sequence. The earliest changes are marked by widespread alveolar and interstitial edema and hemorrhage. Injury to alveolar ducts may be particularly severe. Hyaline membranes, composed of precipitated plasma proteins, fibrin, and necrotic debris, can be seen. The alveolar epithelium may be more extensively damaged than the vascular endothelium, even if the underlying insult is blood-borne. Widespread, local areas of alveolar destruction, particular of type I alveolar epithelial cells, alternate with normal-appearing alveoli. The injured alveolar epithelium is swollen, disorganized, discontinuous, and often lifted off the exposed, but usually intact, basement membranes, which are covered by hyaline membranes. Type II cells are nearly always less severely damaged than type I cells, because their thin squamous cytoplasmic extensions, distant to the nucleus covering the thin side of the alveolar-capillary barrier, are frequently most gravely affected. The interstitium is widened by edema (especially in peribronchovascular cuffs) and may have leukocytes, platelets, red blood cells, fibrin, and debris (especially near the alveolar walls). The microvascular endothelium is often relatively preserved, usually showing little other than irregular focal thickening as a result of cytoplasmic swelling or vacuoles and greater numbers of luminal leukocytes, although frank swelling of endothelial cells may be seen on ultrastructural histology.
The exudative phase is followed by a proliferative phase, which begins within the 5 to 7 days after the onset of injury. The relative contributions of the original insult, repair processes, and effects of therapies to this and subsequent phases are not well known, but some of the abnormalities after the initial exudative phase were related to the effects of traditional modes of mechanical ventilation that used tidal volumes between 12 and 15 mL/kg predicted body weight. In the proliferative phase, some of the edema fluid has been reabsorbed from the air spaces. Fibrin may be prominent in alveoli and interstitium, and there is infiltration with inflammatory cells and fibroblasts. The alveolar epithelium is often cuboidal, made up largely of proliferating type II cells. The air-blood barrier can be thickened by interstitial and epithelial enlargement. The pulmonary vascular bed may be partially or completely disrupted, and structural alterations may reduce its surface area.
A final stage may follow, often about 10 to 14 days after the initial insult, in which fibrotic changes of the alveolar ducts, alveoli, and interstitium predominate: alveoli may be obliterated, alveolar walls coalesced, and functional lung units lost. Less commonly, the lungs show emphysema-like bullous changes. Pulmonary function test results in 5-year survivors of severe increased permeability edema usually return to normal or near-normal values, but exercise limitation and both physical and psychological quality of life are apt to remain compromised.
Mechanisms
The major types of clinical conditions that have been associated with increased permeability edema are listed in Table 62-4 . The most common causes are pneumonia, sepsis, gastric aspiration, and major trauma. The lungs are injured via either the airways or the bloodstream. The exact mechanisms by which diffuse lung injury leads to increased permeability edema have been the subject of intense investigation in humans, animal models, and cellular systems. Human studies have provided descriptive data about events in the air spaces before and after the onset of lung injury. Studies using BAL in patients before and after the onset of diffuse lung damage have shown that there is a major acute inflammatory response that begins before lung injury is clinically recognized, peaks during the first 1 to 3 days of clinical involvement, and then resolves slowly over the next 7 to 14 days in patients who remain intubated. These studies have shown the complexity of the evolving inflammatory responses, which are characterized by the accumulation of acute response cytokines and their inhibitors, oxidants, proteinases and antiproteinases, lipid mediators, growth factors, and collagen precursors involved in the repair process. Extensive efforts have been made to find single biologic markers that predict the onset or the outcome of diffuse parenchymal lung injury, but these have met with only limited success.
|
Hypotheses about mechanisms of lung injury have been tested in animal models and in vitro studies, and several reviews have summarized the findings. The existing animal models do not completely reproduce all of the various aspects of different injuries in humans, in part because human injuries typically evolve over a longer period of time than can be studied in the laboratory. In addition, the lungs of humans are exposed not only to the initial injurious insult but also to the therapies that are used for treatment, such as mechanical ventilation. Experiments with isolated cells have been useful to test specific concepts, but the complexity and redundancy of intact biologic systems are not reproduced in simplified experimental systems. Most experimental work purposely limits a study to a single causative agent; however, this turns the reality of clinical complexity into the simplicity of a single experimental pathway. Increased permeability edema in humans is likely to be caused by interactions between a number of different pathways acting in parallel or in series.
Studies in isolated organs and small animals in which hemodynamic variables are not measured can be difficult to evaluate because indices of lung injury, usually measured by the appearance of markers in lungs, lavage fluid, or perfusate, are not determined solely by the barrier function of the microvasculature. For example, when the vascular endothelium is injured, movement of fluid and protein from the vascular space into the lungs is extremely sensitive to hydrostatic driving pressures and surface area for filtration, and the effects of experimental interventions may be caused by changes in these parameters and not solely by changes in microvascular barrier function. The effects of microvascular driving pressures and surface area can be difficult to evaluate even in large, instrumented animals. Data from experimental animal models suggest that there are at least two broad categories of mechanisms of increased permeability edema: those that are direct (i.e., not requiring intermediary mechanisms, with injury a direct result of contact between an offending substance and lung tissue) and those that are indirect (i.e., requiring the participation of intermediary mechanisms, such as host defenses). These categories overlap because, once the lungs are injured, inflammatory responses may compound the primary mechanism of injury. Three major hypotheses about the mechanism of increased permeability pulmonary edema have been proposed, and are interrelated. A recent review provides specific information on animal models of experimental lung damage and an American Thoracic Society Consensus Conference provided further recommendations.
Diagnosis
The diagnosis of moderately and, especially, severely advanced pulmonary edema, particularly when caused by heart failure, is usually fairly easy. Unraveling the cause of other kinds of pulmonary edema may not be so straightforward, particularly in patients with increased permeability edema.
Clinical Assessment
Definitions
Newcomers interested in the history of catastrophic lung disease should remember the clinical anecdotes that began to surface as far back as the 1950s, 1960s, and 1970s showing that little by little survival from what had been known as “shock lung,” “Danang lung,” and “adult respiratory distress syndrome” represented a gigantic medical-technical-operational breakthrough. Previously, virtually all such egregiously wounded or injured casualties died. (The name adult changed to acute respiratory distress syndrome, but the familiar acronym ARDS remained.) Subsequently, the number of survival miracles steadily increased, but mortality remained high.
Conceptually, ALI comprise a continuum of lung damage ranging from trivial to severe or lethal. For decades and by general agreement, the term “ARDS” has defined the most grievously afflicted victims of the syndrome. In 1988, an “expanded definition” with numerical grading of ARDS was proposed, and 6 years later it was recognized that the term ALI could be “applied to a wide spectrum of this continuum of pathologic process so as to acknowledge and define it” ; moreover, the term ARDS continued to represent the most severe end of the ALI spectrum of damage.
To satisfy clinical and epidemiologic needs, three different definitions of lung damage have been widely used, but each has its shortcomings: the Acute Lung Injury Score, the American-European Consensus Conference (AECC) definition, and a recent revision of the AECC definition termed the “Berlin Definition of ARDS.”
The Lung Injury Score ( Table 62-5 ) provides an assessment of the severity of lung injury, taking into account supportive therapy, such as mechanical ventilation with PEEP and oxygen supplementation. This score is important because not all lung injuries are of equal severity and severity changes over time. In this scoring system, ARDS defines only the most severe injuries (those that yield a score >2.5); milder lung injuries, termed mild-to-moderate, may have a better prognosis and may differ from ARDS in other important aspects. This scoring system has been used widely in clinical research and clinical trials.
1. CHEST RADIOGRAPH SCORE | VALUE |
No alveolar consolidation | 0 |
Alveolar consolidation confined to 1 quadrant | 1 |
Alveolar consolidation confined to 2 quadrants | 2 |
Alveolar consolidation confined to 3 quadrants | 3 |
Alveolar consolidation in all 4 quadrants | 4 |
2. HYPOXEMIA SCORE | |
Pa o 2 /F io 2 ≥ 300 | 0 |
Pa o 2 /F io 2 225–299 | 1 |
Pa o 2 /F io 2 175–224 | 2 |
Pa o 2 /F io 2 100–174 | 3 |
Pa o 2 /F io 2 < 100 | 4 |
3. PEEP SCORE (WHEN VENTILATED) | |
PEEP ≤ 5 cm H 2 O | 0 |
PEEP 6–8 cm H 2 O | 1 |
PEEP 9–11 cm H 2 O | 2 |
PEEP 12–14 cm H 2 O | 3 |
PEEP ≥ 15 cm H 2 O | 4 |
4. RESPIRATORY SYSTEM COMPLIANCE SCORE (WHEN AVAILABLE) | |
Compliance ≥ 80 mL/cm H 2 O | 0 |
Compliance 60–79 mL/cm H 2 O | 1 |
Compliance 40–59 mL/cm H 2 O | 2 |
Compliance 20–39 mL/cm H 2 O | 3 |
Compliance ≤ 19 mL/cm H 2 O | 4 |
The final value is obtained by dividing the aggregate sum by the number of components that were used. | |
SCORE | |
No lung injury | 0 |
Mild to moderate lung injury | 0.1–2.5 |
Severe lung injury (ARDS) | >2.5 |
The American-European Consensus Conference Definition ( Table 62-6 ) has four elements: (1) timing (onset must be “acute”); (2) oxygenation (arterial P o 2 /F io 2 [ fractional concentration of oxygen in inspired gas ] <300 mm Hg, regardless of the level of PEEP, for the diagnosis of ALI to be made, and arterial P o 2 /F io 2 ≤ 200 mm Hg, regardless of PEEP level, for the diagnosis of ARDS to be made); (3) chest radiograph (bilateral opacities seen on frontal view); and (4) pulmonary artery wedge pressure (<18 mm Hg when measured, or no clinical evidence of left atrial hypertension).
TIMING |
Acute |
OXYGENATION (REGARDLESS OF PEEP LEVEL) |
Pa o 2 /F io 2 ≤ 300 = acute lung injury |
Pa o 2 /F io 2 ≤ 200 = acute respiratory distress syndrome |
CHEST RADIOGRAPH |
Bilateral opacities on frontal view |
PULMONARY ARTERY WEDGE PRESSURE |
<18 mm Hg when measured, or |
No clinical evidence of left atrial hypertension |
The third explication, the Berlin Definition, simply subdivides ARDS into three categories of severity: mild, moderate, and severe, based on the arterial P o 2 /F io 2 ratio ( Table 62-7 ). Initially, so-called ancillary variables—severity of chest radiographic findings, minimum PEEP level, respiratory system compliance, and standardized minute volume—were considered for inclusion, but finally discarded. Of interest, the term “acute lung injury” was abandoned. Mortality increases successively as arterial P o 2 /F io 2 ratio worsens in mild, moderate, and severe ARDS.
Mild | Pa o 2 / F io 2 | 200–300 mm Hg |
Moderate | Pa o 2 /F io 2 | 100–199 mm Hg |
Severe ARDS | Pa o 2 / F io 2 | <100 mm Hg |
Another recent method of defining and grading the severity of clinically meaningful lung damage, first in a single center and then in 21 others, is what has been called the Lung Injury Prediction Score , or LIPS, whose goal is to improve the early identification of patients at high risk for development of serious early or impending lung injuries. Both risk factors (predisposing conditions, such as pneumonia, severe sepsis, trauma, and aspiration) and risk modifiers (e.g., alcohol abuse, hypoalbuminemia, and use of supplementary oxygen), have each been graded numerically to yield a combined LIPS value, which has an impressively high negative predictive value (0.96 to 0.98) but a much lower positive predictive value (0.14 to 0.23). A more recent study showed that diffuse alveolar damage was observed in fewer than half the patients having clinical criteria for ARDS, but was more frequent (69%) in those with ARDS lasting more than 72 hours.
The current working definitions all have useful attributes, but fail to link any particular clinical feature to any particular change in the structure or function of the relevant barriers in the lungs or to the degree of pulmonary edema. What defines the ALI-ARDS continuum in a meaningful way is the altered barrier permeability to protein in the lungs, the structural damage to the lung microvascular endothelial and alveolar epithelial barriers, and the consequent excess lung water content. In the future as research studies progress, these clinical definitions may need to be supplemented with biologic markers (see next section) and pathologic findings (when available) to help categorize ALI and ARDS into more specific disease entities.
Symptoms and Signs
The clinical manifestations of pulmonary edema vary with its severity and depend on the underlying pathophysiology and the extent to which excess edema fluid has accumulated in the lungs. Characteristic symptoms comprise dyspnea, cough, and tachypnea. Wheezing, when audible, may present a problem in differential diagnosis, but patients with typical asthma generally do not have other symptoms and signs of congestive heart failure or pulmonary edema. Once alveoli have flooded, the diagnosis of pulmonary edema is not subtle. Patients with alveolar edema usually have severe respiratory distress with tachypnea and cough that is often productive of frothy and sometimes blood-tinged edema fluid. Crackles and rhonchi are heard over the lung fields and wheezing may be present. The patient may be cyanotic if alveolar flooding has seriously compromised gas exchange.
Development of pulmonary edema is often slow and progressive in increased pressure pulmonary edema because the alveoli are protected by the normal safety factors (see Table 62-1 ). In contrast, in increased permeability edema, alveolar flooding and symptoms of respiratory distress often happen rapidly. Edema that develops suddenly (or unexpectedly) sometimes is called “flash pulmonary edema,” which usually pertains to the rapid development of high-pressure edema, after protective safety factors have been surmounted.
Because pulmonary edema is always a sign of an underlying pathologic process, its cause must be identified so that effective therapy can be directed at the underlying problem producing abnormal transvascular fluid and solute flow into the lungs. Increased pressure edema is most often caused by cardiac failure from systolic dysfunction with impaired myocardial contractility and thus is usually accompanied by a history of heart disease; manifestations include signs and symptoms of any of the many causes of chronic and acute congestive heart failure, such as coronary insufficiency, hypertension, valvular heart disease, and severe volume expansion. Elevated jugular venous pressure, cardiac enlargement, gallop rhythms, heart murmurs, arrhythmias, large tender liver, and peripheral edema almost always suggest an underlying abnormality of cardiac function. However, pulmonary edema may be the only manifestation of silent myocardial infarction or diastolic dysfunction of the left ventricle.
History and physical examination may also be helpful in differentiating between increased pressure and increased permeability pulmonary edema, because most patients in the latter category usually do not have signs or symptoms of underlying cardiac disease. The cause of increased permeability edema may be suggested by a history of exposure (e.g., to toxic gases or chemicals, near-drowning, drug ingestion, trauma), the clinical setting (e.g., sepsis, pneumonia, emesis, seizures, pancreatitis), or the physical findings (e.g., chest trauma, long bone fractures, coma, shock). Because infections, including the sepsis syndrome, are the leading causes of increased permeability edema in patients, a thorough search must be made for signs and symptoms of infection. Pulmonary and intra-abdominal sources are the most common sites of involvement, and all patients should be examined carefully, with special attention being paid to abdominal, rectal, and pelvic examinations.
Diagnostic Studies
Laboratory and other diagnostic studies are often helpful but, by the time many of the results are found to be abnormal, the diagnosis is usually obvious. Appropriate cultures for microorganisms and toxicology screens of blood and urine are useful in identifying underlying causes of increased permeability edema. Examination of sputum or tracheal aspirate, bronchoscopy with protected-specimen brushing, or mini-BAL are all useful in diagnosing pneumonia in ventilated patients, even those who are being treated with antimicrobial drugs. Lung biopsy can sometimes provide a specific diagnosis in critically ill patients, but the results often are not helpful because lung injuries caused by diverse underlying conditions have similar histologic appearances and because specific therapies may not be available.
In the special case of pulmonary edema suspected to be caused by saltwater near-drowning, measurement of plasma magnesium level can help determine whether a patient has aspirated or swallowed seawater, or both. Severe hypermagnesemia has been reported following aspiration of ordinary seawater and, especially, highly concentrated saltwater from the Dead Sea.
Chest Radiographs
The plain chest radiograph is the most practical laboratory study available for the detection of pulmonary edema. Disadvantages are that chest radiographs are insensitive to small changes in lung water and are only semiquantitative. An additional limitation is that chest radiographs are not consistently helpful in distinguishing increased pressure edema from increased permeability edema. These disadvantages are offset by the advantages that chest radiographs are noninvasive, inexpensive, easily repeatable, readily available, and free of serious side effects (apart from a small amount of radiation).
Before alveolar flooding, plain chest radiographs typically show distended vascular shadows (particularly in the upper lung fields), enlargement and loss of definition of hilar structures, development of septal lines (Kerley lines) ( Fig. 62-5 ; , loss of peribronchial and perivascular definition or cuffing) ( Fig. 62-6 ), and perihilar haze indicating the presence of interstitial pulmonary edema. Acinar shadows, often confluent and creating irregular, patchy increases in lung density that obscure vascular markings, indicate the presence of alveolar edema. Air bronchograms may be observed in severe edema. Because the radiographic signs of interstitial and alveolar edema are determined by gas and blood volumes and their distribution in the lungs in addition to the presence of edema, the recognition and quantitation of edema are not precise, and the radiographic appearance of edema is strongly influenced by the lung volume at the time the film is made. The chest radiograph score is an integral part of the Lung Injury Score and the revised Berlin Definition, but the interpretation of chest radiographs is not well standardized and significant interobserver variations have been reported. One recent approach for scoring the chest radiograph and accounting for atelectasis correlated well with lung weight in lungs that were studied from brain-dead potential organ donors.
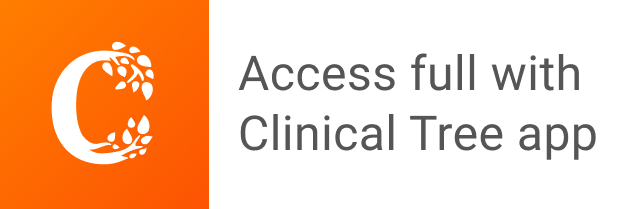