Introduction
The pulmonary circulation is interposed between the right and left ventricles with the following primary functions: (1) to deliver the entire cardiac output under low pressure from the right ventricle to the pulmonary microvessels and, in the process, to exchange carbon dioxide for oxygen across the alveolar-capillary membrane; (2) to act as a source of production, release, and processing of humoral mediators; and (3) to serve as a barrier to the exchange of fluid and solutes and thus maintain lung fluid balance. The morphologic characteristics of the pulmonary circulation are ideally adapted for these functions. Nearly the entire cardiac output is brought into contact with alveolar gas at the 1- to 2-µm-thick alveolar-capillary membrane for approximately 0.75 to 1 second. This juxtaposition of capillaries with alveoli provides the vast surface area needed for effective gas exchange: approximately 70 m 2 (two thirds the area of a tennis court). The structural arrangement is such that the distance through which oxygen and carbon dioxide must diffuse between gas and blood is approximately one tenth the distance of diffusion in peripheral tissues. (Additional information about the anatomy of the pulmonary circulation is found in Chapter 1 , and about the physiologic factors that govern the distribution of blood flow in Chapter 4 .)
The pulmonary circulation has important additional functions beyond its role in gas exchange. The microvessels exchange solutes and water, and the mechanisms regulating the balance of fluid and solutes in extravascular spaces of the lung are critical to the understanding of the pathophysiology of pulmonary edema (see section “Pathogenesis of Pulmonary Edema” and Chapter 62 ). The pulmonary vascular endothelium, the monolayer of cells that lines all vessels, is a multidimensional tissue whose specialized functions include direct lung vascular barrier regulation, participation in the initiation and resolution of inflammatory responses and the processing of mediators before delivery to the systemic circulation.
Anatomy
The pulmonary circulation begins at the pulmonary valve, marking the vascular exit from the right side of the heart, and extends to the orifices of the pulmonary veins in the wall of the left atrium, which marks the entrance into the left side of the heart. The pulmonary circulation includes the pulmonary trunk (also called the “right ventricular outflow tract”), the right and left main pulmonary arteries and their lobar branches, intrapulmonary arteries, large elastic arteries, small muscular arteries, arterioles, capillaries, venules, and large pulmonary veins. Because of this heterogeneity and differences in physiologic behavior, the vessels of the pulmonary circulation are subdivided on a functional basis into extra-alveolar vessels and alveolar vessels . In addition, the small vessels that participate in liquid and solute exchange are often collectively termed the “pulmonary microcirculation.” The anatomic boundaries of the extra-alveolar and alveolar vessels and the microcirculation are undefined and likely depend on conditions such as lung volume and levels of intrapleural and interstitial pressures.
Additional information on the anatomy of the pulmonary circulation as well as the bronchial circulation can be found in Chapter 1 .
General Design
The pulmonary trunk arises from the infundibulum of the right ventricle through the orifice of the pulmonary valve. The trunk, which is approximately 3 cm in diameter and 5 cm in length, lies entirely within the pericardium, as does the adjacent ascending aorta. The pulmonary trunk passes upward and backward into the concavity of the aortic arch, where it divides into the two main pulmonary arteries.
The right main pulmonary artery is slightly larger and longer than the left main pulmonary artery , and both vessels show little variation in either position or mode of branching. The right main artery divides into two branches: a larger lower branch that supplies the right middle and lower lobes, and a smaller upper branch that supplies the upper lobe. On the left the main artery lies above the main bronchus until the first branch arises and subsequently traverses in a downward direction behind the bronchus. The arterial branches supplying the lobes of both the right and left lungs, in contrast, show considerable variation. The pulmonary arteries and bronchi are enclosed in the same connective tissue sheath and generally branch together until reaching the smallest units: the alveoli and capillaries. The pulmonary veins are also enveloped in connective tissue sheaths that are distinct from those enclosing the arteries and bronchi.
The pulmonary arterial circulation has two sets of branches: the conventional arteries that accompany airways, and supernumerary arteries that travel alone and are commonly of smaller dimensions. The supernumerary arteries are entirely intrapulmonary, emerging from the main arterial channels at approximately right angles as far in the periphery as the end of respiratory bronchioles. These branches provide approximately 25% of the total cross-sectional area of the pulmonary arterial bed near the hilum and approximately 40% of the total cross-sectional area of the peripheral pulmonary arterial bed. The supernumerary arteries that are present at birth are mainly those that lead to terminal respiratory units, the structures distal to terminal bronchioles that participate in gas exchange (respiratory bronchioles, alveolar ducts, and alveoli). Extensive growth of conventional and supernumerary branches accompanies the development of alveolar ducts and alveoli during the first 18 months of life. Whereas the appearance of conventional arteries virtually ceases at 18 months, supernumerary arteries continue to increase in number up to approximately age 8 years as new alveoli are formed. Supernumerary vessels undoubtedly serve as an auxiliary arterial supply to the capillary beds of the terminal respiratory units and thus constitute a critical source of collateral blood flow to sites of gas exchange.
The pulmonary vascular resistance is approximately one tenth the systemic peripheral vascular resistance. This unique low-resistance characteristic of the pulmonary circulation is the result of specialized morphologic features of pulmonary arteries and veins combined with a normally low vascular tone. Both pulmonary arteries and veins have significantly less smooth muscle content than do vessels of the same diameter in other organs, and the smooth muscle is distributed less evenly than in systemic microvessels. Pulmonary arteries exhibit more smooth muscle than pulmonary veins and represent the main sites of constriction in response to vasoactive mediators.
In humans the larger pulmonary arteries (>1 to 2 mm in diameter) are largely elastic. The elastic pulmonary arteries contain distinctive layers of elastic fibers embedded in a coat of smooth muscle cells. In fact, the pulmonary artery trunk, its main branches, and all extra-alveolar pulmonary arteries are of the elastic type. Arteries gradually become more muscular at diameters of greater than 2 mm followed by progressively less muscularization as diameter decreases to below 100 µm. The smooth muscle is unevenly distributed in the medial layer of the vessel wall in a way that is distinct from the thick circumferential distribution of smooth muscle in systemic arterioles. The muscular pulmonary arteries exhibit a thin medial layer of muscle embedded between well-delimited internal and external elastic laminae. Muscular pulmonary arteries lie within lung lobules and hence accompany the bronchioles. Although these vessels are designated by their muscular elements, the thickness of the muscle layer does not exceed approximately 5% of the external diameter of the vessel; any increase above this value connotes a pathologic state, as is clinically associated with conditions characterized by the presence of pulmonary arterial hypertension. As the smooth muscle content decreases in these vessels, the smooth muscle assumes a spiral orientation so that in a cross section of the vessel, the smooth muscle content is observed in only a portion of the vessel wall. These pulmonary arterioles are the terminal branches of the pulmonary arterial system. At their origin from muscular arteries, pulmonary arterioles contain a partial layer of muscle that gradually disappears until the vessel wall consists of only the endothelial monolayer and an elastic lamina. Pulmonary arterioles supply alveolar ducts and alveoli. In vessels smaller than 30 µm in diameter, smooth muscle is virtually absent. Chronic exposure to hypoxia causes extensive vascular remodeling, especially with significant increase in smooth muscle in small arteries. There is considerable species variability in pulmonary artery smooth muscle content and in the pulmonary hypertensive response to hypoxia.
Pulmonary veins have thinner walls than arteries because the muscular layer is not as well developed. Like the arterial system, the venous system is composed of both conventional and supernumerary veins. Small intrapulmonary venules successively unite to form increasingly larger veins, and finally a single lobar vein emerges from each lobe. Because the right upper and right middle lobar veins generally join together, the venous drainage from each lung terminates in a superior pulmonary vein and an inferior pulmonary vein . These four pulmonary veins then enter the left atrium through orifices in the upper posterior part of the chamber wall, although occasionally the two left veins join and enter through a common opening. The pulmonary vascular bed consists of a set of highly distensible vessels, a unique feature of this circulation in that pulmonary vessels are approximately seven times more compliant than peripheral systemic arteries due to the reduced smooth muscle content, fewer elastin and collagen fibers, and the lack of tissue surrounding the small vessels. The resistance and distensibility functions of a pulmonary vessel overlap in the same vessel. Thus pulmonary vessels are able to accommodate relatively large increases in blood volume (as in exercise) in relation to same-sized systemic arteries and serve as an important blood volume reservoir. Smooth muscle cells are intercalated with the elastin and collagen fibers, thereby enabling reflex contraction of smooth muscle cells to vary the distensibility of the pulmonary vessels. In the microvasculature, pericytes, polymorphic and multifunctional cells that line the basal surface of endothelial cells, are involved in the production of the microvessel basal lamina and contribute to the contractile regulation of lung capillary permeability.
Pulmonary vessels are innervated by cholinergic and sympathetic fibers, although the extent of the innervation is species specific and may vary from animal to animal. In comparison with peripheral vessels, the innervation pattern is sparse, with innervation most evident in the branching-off point of pulmonary arteries. Sympathetic and parasympathetic efferents are most prominent in small bronchioles and arterioles of the bronchial circulation, in contrast to pulmonary vessels, although the exact function remains incompletely defined. Recent studies have shown the densest innervation in the vessels in the bronchial walls, and that synaptophysin-immunoreactive terminals are formed by efferent axons of neurons arising from the intrapulmonary parasympathetic ganglia. Furthermore, sympathetic pulmonary vascular neurons appear to be activated via arterial chemoreceptors in response to low P o 2 .
Bronchial Circulation
A separate systemic circulation supplies blood flow to the airways from the carina to the terminal bronchioles. In addition, bronchial arteries provide nutritive flow to the lower trachea, airway nerves, and lymph nodes. The drainage of bronchial vessels into the pulmonary circulation and the large veins has a complex arrangement ( eFig. 6-1 ). Interconnections have been demonstrated between bronchial vessels and precapillary, capillary, and postcapillary vessels of the pulmonary circulation. Despite the fact that the normal adult lung remains viable without the bronchial circulation (as well as in the absence of innervation), as is the case in the transplanted lung, bronchial blood flow is critical in the lung development in the fetus and contributes to gas exchange in the presence of congenital cardiac anomalies. There is a striking increase in the size and number of bronchial arteries (due to angiogenesis) in lung disorders such as pulmonary fibrosis, lung carcinoma, and disorders characterized by pulmonary vascular occlusion. Neovascularization of the systemic circulation into the lung after pulmonary artery obstruction is now well recognized in multiple species.
There is considerable variation in the number and origin of the bronchial arteries in the human adult. One large cadaveric study found the majority of bronchial arteries arising directly from the aorta. Over 40% of cadavers had two arteries branching to the left lung and one artery to the right lung. Occasionally the right bronchial artery originates from the first right intercostal artery. Variable numbers of smaller minor branches emerged from vessels in and near the mediastinum and crossed into each lung. Upon entering the lung, bronchial arteries invest in connective tissue surrounding the bronchi and begin branching with two or three branches anastomosing to form a peribronchial plexus with an elongated and irregular mesh and accompany each subdivision of the conducting airways.
Blood flow to the lung via the bronchial circulation is low, presumed to be less than 3% of the cardiac output, but has never been measured accurately in humans. Canine studies indicate that bronchial blood flow to the left lung is approximately 1% of the cardiac output, with approximately 50% of this flow directed to the lung parenchyma and the remainder to the trachea and bronchi. If bronchial blood flow in humans is similar to canine findings, total bronchial blood flow estimates to both lungs would be approximate 1% to 2% of the cardiac output.
Venous blood from capillaries supplied by bronchial arteries returns to the heart by two different pathways (see eFig. 6-1 ). True bronchial veins are found only at the hilum; formed from tributaries that originate around the lobar and segmental bronchi and from branches from the pleura in the neighborhood of the hilum. Bronchial venous blood empties into the azygos, hemiazygos, or intercostal veins and flows into the right atrium. Veins that originate from bronchial capillaries within the lungs unite to form venous tributaries that join the pulmonary veins with these communicating vessels termed the “bronchopulmonary veins.” Blood leaving the capillary bed around terminal bronchioles flows through anastomoses with alveolar capillaries, and the mixture returns to the left atrium via pulmonary veins.
The distribution of bronchial arterial inflow between the two available venous outflow pathways is unknown in humans, with tentative conclusions drawn from the technically demanding studies in experimental animals. These indicate that approximately 25% to 33% of the bronchial arterial supply returns ultimately to the right atrium via bronchial veins, and 67% to 75% flows into the left atrium via pulmonary veins.
Controversy still exists concerning the presence and significance of bronchopulmonary arterial anastomoses (i.e., direct vascular connections between pulmonary arteries and bronchial arteries). Available evidence suggests that bronchopulmonary arterial anastomoses exist, are readily identifiable in neonatal lungs but are infrequent in normal lungs, and increase considerably in certain lung pathologic conditions.
Blood-Gas Interface
The pulmonary capillaries form an extensive network interwoven with a meshwork of parenchymatous connective tissue (fine collagen and elastin fibers) in the interalveolar septa , the walls that separate adjacent alveoli. The capillary bed has been described either as a hexagonal meshwork of cylinders only minimally longer than its diameter, or as a sheet with the two sides periodically connected by septal tissue posts. These two models are useful for theoretical analysis of flow in pulmonary capillaries, but each represents simplification of a complex capillary network. Capillary perfusion begins when intracapillary pressure begins to exceed alveolar pressure and additional capillaries are recruited, with further increases in capillary pressure depending on the tension in the alveolar wall, whether imposed by positive airway pressure or by gravity when the lung is suspended in an intact thorax.
Pulmonary capillaries, composed of capillary endothelium contiguously arranged to form a thin vascular tube, weave through the interstitial space of the interalveolar septum, facing first one alveolus and then another, thereby crossing several alveoli. Both the endothelium and the neighboring type I and type II alveolar epithelium rest on individual basement membranes, which appear to be fused over more than 50% of the capillary perimeter, thereby forming the thin portion of the alveolar-capillary septum. This is an ideal site for gas exchange because of the maximum surface area available for gas exchange combined with an extremely short diffusion distance. The thin septum consists of connective tissue elements (primarily collagens I and IV) that provide structural support. In the remaining half of the capillary perimeter, the endothelial and epithelial basement membranes are separated to form an interstitial space (i.e., the thick portion of the alveolar septum, which represents the primary site of transcapillary fluid and solute exchange and consists of a variety of collagen fibers, elastin, and proteoglycans). The existing barriers from the air space to the vessel lumen are the alveolar epithelium , basement membranes of the epithelium and endothelium, the interstitial space that exists within the thick septum but not the thin septum, and the endothelium .
Imaging the Pulmonary Circulation
Advances in techniques to image the pulmonary circulation in recent years serve as invaluable tools to evaluate patients with potential pulmonary vascular abnormalities or diseases. Although computed tomography is one useful modality for these purposes, magnetic resonance imaging (MRI) is recognized as the most effective approach currently in use by virtue of its ability to visualize vessels regardless of orientation and to provide functional in addition to anatomic information. In addition, MRI, unlike computed tomography, does not require administration of potentially toxic radiation or contrast agents.
Two MRI techniques of high utility are contrast-enhanced magnetic resonance angiography and phase-contrast magnetic resonance velocity mapping. Magnetic resonance angiography relies on the intravenous administration of gadolinium to obtain rapid and detailed imaging of lung vascular anatomy with a total imaging time of less than 20 seconds, often acquiring relatively high resolution three-dimensional images within 3 to 4 seconds. This allows for pulmonary vasculature visualization even in significantly dyspneic patients unable to hold their breath for a prolonged period of time. Relying on a pixel-by-pixel analysis of signal intensity-time course curves, magnetic resonance angiography is also capable of providing functional data, including quantitation of regional perfusion in terms of flow, mean transit time, and volume throughout the lungs. Alternatively, phase-contrast MRI provides similar functional assessments derived from measurements of phase shifts of moving nuclei relative to stationary nuclei. Although precise measurements can most reliably be obtained from the central vessels using this technique, flow patterns can be accurately determined throughout the lung vasculature, including the peripheral veins, and, in general, phase-contrast MRI flow measurements provide greater than 95% accuracy.
MRI of the pulmonary circulation offers significant clinical applications. For example, MRI provides full visualization of anomalous pulmonary venous connections (representing approximately 2% of congenital heart diseases) and assessment of hemodynamic parameters. Thoracic MRI has improved its potential in pulmonary thromboembolism, whereas use of multidetector-row CT has been used in quantifying bilateral bronchial narrowing. Separately, magnetic resonance angiography is now the optimal modality to evaluate pulmonary vein stenosis, surpassing the sensitivity of echocardiography and allowing assessment of stenotic lesions in addition to the collateral circulation. A number of other potential clinical applications with respect to the pulmonary vasculature have shown high promise and continue to be the focus of investigation, including the evaluation of patients with chronic thromboembolic disease, arteriovenous malformations, and pulmonary vasculitis.
General Design
The pulmonary trunk arises from the infundibulum of the right ventricle through the orifice of the pulmonary valve. The trunk, which is approximately 3 cm in diameter and 5 cm in length, lies entirely within the pericardium, as does the adjacent ascending aorta. The pulmonary trunk passes upward and backward into the concavity of the aortic arch, where it divides into the two main pulmonary arteries.
The right main pulmonary artery is slightly larger and longer than the left main pulmonary artery , and both vessels show little variation in either position or mode of branching. The right main artery divides into two branches: a larger lower branch that supplies the right middle and lower lobes, and a smaller upper branch that supplies the upper lobe. On the left the main artery lies above the main bronchus until the first branch arises and subsequently traverses in a downward direction behind the bronchus. The arterial branches supplying the lobes of both the right and left lungs, in contrast, show considerable variation. The pulmonary arteries and bronchi are enclosed in the same connective tissue sheath and generally branch together until reaching the smallest units: the alveoli and capillaries. The pulmonary veins are also enveloped in connective tissue sheaths that are distinct from those enclosing the arteries and bronchi.
The pulmonary arterial circulation has two sets of branches: the conventional arteries that accompany airways, and supernumerary arteries that travel alone and are commonly of smaller dimensions. The supernumerary arteries are entirely intrapulmonary, emerging from the main arterial channels at approximately right angles as far in the periphery as the end of respiratory bronchioles. These branches provide approximately 25% of the total cross-sectional area of the pulmonary arterial bed near the hilum and approximately 40% of the total cross-sectional area of the peripheral pulmonary arterial bed. The supernumerary arteries that are present at birth are mainly those that lead to terminal respiratory units, the structures distal to terminal bronchioles that participate in gas exchange (respiratory bronchioles, alveolar ducts, and alveoli). Extensive growth of conventional and supernumerary branches accompanies the development of alveolar ducts and alveoli during the first 18 months of life. Whereas the appearance of conventional arteries virtually ceases at 18 months, supernumerary arteries continue to increase in number up to approximately age 8 years as new alveoli are formed. Supernumerary vessels undoubtedly serve as an auxiliary arterial supply to the capillary beds of the terminal respiratory units and thus constitute a critical source of collateral blood flow to sites of gas exchange.
The pulmonary vascular resistance is approximately one tenth the systemic peripheral vascular resistance. This unique low-resistance characteristic of the pulmonary circulation is the result of specialized morphologic features of pulmonary arteries and veins combined with a normally low vascular tone. Both pulmonary arteries and veins have significantly less smooth muscle content than do vessels of the same diameter in other organs, and the smooth muscle is distributed less evenly than in systemic microvessels. Pulmonary arteries exhibit more smooth muscle than pulmonary veins and represent the main sites of constriction in response to vasoactive mediators.
In humans the larger pulmonary arteries (>1 to 2 mm in diameter) are largely elastic. The elastic pulmonary arteries contain distinctive layers of elastic fibers embedded in a coat of smooth muscle cells. In fact, the pulmonary artery trunk, its main branches, and all extra-alveolar pulmonary arteries are of the elastic type. Arteries gradually become more muscular at diameters of greater than 2 mm followed by progressively less muscularization as diameter decreases to below 100 µm. The smooth muscle is unevenly distributed in the medial layer of the vessel wall in a way that is distinct from the thick circumferential distribution of smooth muscle in systemic arterioles. The muscular pulmonary arteries exhibit a thin medial layer of muscle embedded between well-delimited internal and external elastic laminae. Muscular pulmonary arteries lie within lung lobules and hence accompany the bronchioles. Although these vessels are designated by their muscular elements, the thickness of the muscle layer does not exceed approximately 5% of the external diameter of the vessel; any increase above this value connotes a pathologic state, as is clinically associated with conditions characterized by the presence of pulmonary arterial hypertension. As the smooth muscle content decreases in these vessels, the smooth muscle assumes a spiral orientation so that in a cross section of the vessel, the smooth muscle content is observed in only a portion of the vessel wall. These pulmonary arterioles are the terminal branches of the pulmonary arterial system. At their origin from muscular arteries, pulmonary arterioles contain a partial layer of muscle that gradually disappears until the vessel wall consists of only the endothelial monolayer and an elastic lamina. Pulmonary arterioles supply alveolar ducts and alveoli. In vessels smaller than 30 µm in diameter, smooth muscle is virtually absent. Chronic exposure to hypoxia causes extensive vascular remodeling, especially with significant increase in smooth muscle in small arteries. There is considerable species variability in pulmonary artery smooth muscle content and in the pulmonary hypertensive response to hypoxia.
Pulmonary veins have thinner walls than arteries because the muscular layer is not as well developed. Like the arterial system, the venous system is composed of both conventional and supernumerary veins. Small intrapulmonary venules successively unite to form increasingly larger veins, and finally a single lobar vein emerges from each lobe. Because the right upper and right middle lobar veins generally join together, the venous drainage from each lung terminates in a superior pulmonary vein and an inferior pulmonary vein . These four pulmonary veins then enter the left atrium through orifices in the upper posterior part of the chamber wall, although occasionally the two left veins join and enter through a common opening. The pulmonary vascular bed consists of a set of highly distensible vessels, a unique feature of this circulation in that pulmonary vessels are approximately seven times more compliant than peripheral systemic arteries due to the reduced smooth muscle content, fewer elastin and collagen fibers, and the lack of tissue surrounding the small vessels. The resistance and distensibility functions of a pulmonary vessel overlap in the same vessel. Thus pulmonary vessels are able to accommodate relatively large increases in blood volume (as in exercise) in relation to same-sized systemic arteries and serve as an important blood volume reservoir. Smooth muscle cells are intercalated with the elastin and collagen fibers, thereby enabling reflex contraction of smooth muscle cells to vary the distensibility of the pulmonary vessels. In the microvasculature, pericytes, polymorphic and multifunctional cells that line the basal surface of endothelial cells, are involved in the production of the microvessel basal lamina and contribute to the contractile regulation of lung capillary permeability.
Pulmonary vessels are innervated by cholinergic and sympathetic fibers, although the extent of the innervation is species specific and may vary from animal to animal. In comparison with peripheral vessels, the innervation pattern is sparse, with innervation most evident in the branching-off point of pulmonary arteries. Sympathetic and parasympathetic efferents are most prominent in small bronchioles and arterioles of the bronchial circulation, in contrast to pulmonary vessels, although the exact function remains incompletely defined. Recent studies have shown the densest innervation in the vessels in the bronchial walls, and that synaptophysin-immunoreactive terminals are formed by efferent axons of neurons arising from the intrapulmonary parasympathetic ganglia. Furthermore, sympathetic pulmonary vascular neurons appear to be activated via arterial chemoreceptors in response to low P o 2 .
Bronchial Circulation
A separate systemic circulation supplies blood flow to the airways from the carina to the terminal bronchioles. In addition, bronchial arteries provide nutritive flow to the lower trachea, airway nerves, and lymph nodes. The drainage of bronchial vessels into the pulmonary circulation and the large veins has a complex arrangement ( eFig. 6-1 ). Interconnections have been demonstrated between bronchial vessels and precapillary, capillary, and postcapillary vessels of the pulmonary circulation. Despite the fact that the normal adult lung remains viable without the bronchial circulation (as well as in the absence of innervation), as is the case in the transplanted lung, bronchial blood flow is critical in the lung development in the fetus and contributes to gas exchange in the presence of congenital cardiac anomalies. There is a striking increase in the size and number of bronchial arteries (due to angiogenesis) in lung disorders such as pulmonary fibrosis, lung carcinoma, and disorders characterized by pulmonary vascular occlusion. Neovascularization of the systemic circulation into the lung after pulmonary artery obstruction is now well recognized in multiple species.
There is considerable variation in the number and origin of the bronchial arteries in the human adult. One large cadaveric study found the majority of bronchial arteries arising directly from the aorta. Over 40% of cadavers had two arteries branching to the left lung and one artery to the right lung. Occasionally the right bronchial artery originates from the first right intercostal artery. Variable numbers of smaller minor branches emerged from vessels in and near the mediastinum and crossed into each lung. Upon entering the lung, bronchial arteries invest in connective tissue surrounding the bronchi and begin branching with two or three branches anastomosing to form a peribronchial plexus with an elongated and irregular mesh and accompany each subdivision of the conducting airways.
Blood flow to the lung via the bronchial circulation is low, presumed to be less than 3% of the cardiac output, but has never been measured accurately in humans. Canine studies indicate that bronchial blood flow to the left lung is approximately 1% of the cardiac output, with approximately 50% of this flow directed to the lung parenchyma and the remainder to the trachea and bronchi. If bronchial blood flow in humans is similar to canine findings, total bronchial blood flow estimates to both lungs would be approximate 1% to 2% of the cardiac output.
Venous blood from capillaries supplied by bronchial arteries returns to the heart by two different pathways (see eFig. 6-1 ). True bronchial veins are found only at the hilum; formed from tributaries that originate around the lobar and segmental bronchi and from branches from the pleura in the neighborhood of the hilum. Bronchial venous blood empties into the azygos, hemiazygos, or intercostal veins and flows into the right atrium. Veins that originate from bronchial capillaries within the lungs unite to form venous tributaries that join the pulmonary veins with these communicating vessels termed the “bronchopulmonary veins.” Blood leaving the capillary bed around terminal bronchioles flows through anastomoses with alveolar capillaries, and the mixture returns to the left atrium via pulmonary veins.
The distribution of bronchial arterial inflow between the two available venous outflow pathways is unknown in humans, with tentative conclusions drawn from the technically demanding studies in experimental animals. These indicate that approximately 25% to 33% of the bronchial arterial supply returns ultimately to the right atrium via bronchial veins, and 67% to 75% flows into the left atrium via pulmonary veins.
Controversy still exists concerning the presence and significance of bronchopulmonary arterial anastomoses (i.e., direct vascular connections between pulmonary arteries and bronchial arteries). Available evidence suggests that bronchopulmonary arterial anastomoses exist, are readily identifiable in neonatal lungs but are infrequent in normal lungs, and increase considerably in certain lung pathologic conditions.
Blood-Gas Interface
The pulmonary capillaries form an extensive network interwoven with a meshwork of parenchymatous connective tissue (fine collagen and elastin fibers) in the interalveolar septa , the walls that separate adjacent alveoli. The capillary bed has been described either as a hexagonal meshwork of cylinders only minimally longer than its diameter, or as a sheet with the two sides periodically connected by septal tissue posts. These two models are useful for theoretical analysis of flow in pulmonary capillaries, but each represents simplification of a complex capillary network. Capillary perfusion begins when intracapillary pressure begins to exceed alveolar pressure and additional capillaries are recruited, with further increases in capillary pressure depending on the tension in the alveolar wall, whether imposed by positive airway pressure or by gravity when the lung is suspended in an intact thorax.
Pulmonary capillaries, composed of capillary endothelium contiguously arranged to form a thin vascular tube, weave through the interstitial space of the interalveolar septum, facing first one alveolus and then another, thereby crossing several alveoli. Both the endothelium and the neighboring type I and type II alveolar epithelium rest on individual basement membranes, which appear to be fused over more than 50% of the capillary perimeter, thereby forming the thin portion of the alveolar-capillary septum. This is an ideal site for gas exchange because of the maximum surface area available for gas exchange combined with an extremely short diffusion distance. The thin septum consists of connective tissue elements (primarily collagens I and IV) that provide structural support. In the remaining half of the capillary perimeter, the endothelial and epithelial basement membranes are separated to form an interstitial space (i.e., the thick portion of the alveolar septum, which represents the primary site of transcapillary fluid and solute exchange and consists of a variety of collagen fibers, elastin, and proteoglycans). The existing barriers from the air space to the vessel lumen are the alveolar epithelium , basement membranes of the epithelium and endothelium, the interstitial space that exists within the thick septum but not the thin septum, and the endothelium .
Imaging the Pulmonary Circulation
Advances in techniques to image the pulmonary circulation in recent years serve as invaluable tools to evaluate patients with potential pulmonary vascular abnormalities or diseases. Although computed tomography is one useful modality for these purposes, magnetic resonance imaging (MRI) is recognized as the most effective approach currently in use by virtue of its ability to visualize vessels regardless of orientation and to provide functional in addition to anatomic information. In addition, MRI, unlike computed tomography, does not require administration of potentially toxic radiation or contrast agents.
Two MRI techniques of high utility are contrast-enhanced magnetic resonance angiography and phase-contrast magnetic resonance velocity mapping. Magnetic resonance angiography relies on the intravenous administration of gadolinium to obtain rapid and detailed imaging of lung vascular anatomy with a total imaging time of less than 20 seconds, often acquiring relatively high resolution three-dimensional images within 3 to 4 seconds. This allows for pulmonary vasculature visualization even in significantly dyspneic patients unable to hold their breath for a prolonged period of time. Relying on a pixel-by-pixel analysis of signal intensity-time course curves, magnetic resonance angiography is also capable of providing functional data, including quantitation of regional perfusion in terms of flow, mean transit time, and volume throughout the lungs. Alternatively, phase-contrast MRI provides similar functional assessments derived from measurements of phase shifts of moving nuclei relative to stationary nuclei. Although precise measurements can most reliably be obtained from the central vessels using this technique, flow patterns can be accurately determined throughout the lung vasculature, including the peripheral veins, and, in general, phase-contrast MRI flow measurements provide greater than 95% accuracy.
MRI of the pulmonary circulation offers significant clinical applications. For example, MRI provides full visualization of anomalous pulmonary venous connections (representing approximately 2% of congenital heart diseases) and assessment of hemodynamic parameters. Thoracic MRI has improved its potential in pulmonary thromboembolism, whereas use of multidetector-row CT has been used in quantifying bilateral bronchial narrowing. Separately, magnetic resonance angiography is now the optimal modality to evaluate pulmonary vein stenosis, surpassing the sensitivity of echocardiography and allowing assessment of stenotic lesions in addition to the collateral circulation. A number of other potential clinical applications with respect to the pulmonary vasculature have shown high promise and continue to be the focus of investigation, including the evaluation of patients with chronic thromboembolic disease, arteriovenous malformations, and pulmonary vasculitis.
Pulmonary Hemodynamics
Pulmonary Vascular Pressures
Pressure and flow are highly pulsatile throughout the pulmonary circulation. Although the pressure pulsatility decreases across the pulmonary circuit, the pulsatile nature of the flow persists on the venous side. Pulmonary artery pressure (P pa ) is normally approximately 25 mm Hg during systole and 9 mm Hg during diastole. Relative to systemic arterial pressure, P pa is low, and hydrostatic pressure differences due to gravity result in a substantial difference in vascular pressure from the top to the bottom of the lung. If the pulmonary artery is considered to be a column of blood approximately 25 cm high, there will be a 25 cm H 2 O (or 18 mm Hg) P pa increase from the bottom to the top of the lung (1 mm Hg pressure = 1.36 cm H 2 O pressure). This pressure difference results in a nonuniform distribution of blood flow, as discussed subsequently in the “Regional Distribution of Pulmonary Perfusion” section.
P pa is measured by inserting a cardiac catheter or a balloon-tipped flotation catheter into the pulmonary artery. Inflating the balloon leads to advancement (“floating”) of the catheter ( Fig. 6-1 ) until it “wedges” and occludes a peripheral pulmonary artery. With the balloon inflated the pressure measured in the tip of the catheter is called the pulmonary wedge pressure (P pw ). This procedure effectively extends the static fluid within the catheter lumen into the vascular bed, and the measured pressure is thus at the site where this extended column next joins a vessel in which blood is flowing. The wedge pressure (normally 5 to 10 mm Hg) is an estimate of the vascular pressure at the point of confluence of pulmonary veins and hence reflects left atrial pressure (P la ).

Changes in pressure distal to the confluence of the pulmonary veins, such as that induced by constriction of the pulmonary venules, can alter the relationship between P la and P pw . Also, the precise location of the catheter tip in the lung influences the measurement of P pw ( Fig. 6-2 ). Zone 1 is the region of the lung in which the alveolar pressure (Palv) is greater than P pa , which is greater than the pulmonary venous pressure (P pv ), and therefore there is minimal blood flow through the alveolar vessels. Zone 2 is where P pa is greater than Palv, which is greater than P pv , and therefore flow increases linearly as one moves down the lung. Positioning the catheter in the upper lung (in zone 1 or 2) results in a P pw different from P la because higher alveolar pressures occlude the fluid column. Under these conditions, P pw provides an incorrect measurement of pulmonary vascular outflow pressure. A catheter wedged in zone 3, where P pa is greater than P pv , which is greater than Palv, more accurately reflects the P la . Various algorithms have been proposed to validate measurements of P pw .

Pulmonary Vascular Resistance
Pulmonary vascular resistance (PVR) is calculated by the following equation:
PVR = PPA − PLA Q ˙ T
where is cardiac output, P pa is mean pulmonary artery (inflow) pressure, and P la is mean left atrial (outflow) pressure, which is often estimated by
. PVR is expressed in units of mm Hg/L/min or in dyne•sec•cm −5 (to convert units to dyne•sec•cm −5 , PVR in mm Hg/L/min is multiplied by 1332). The normal PVR value is approximately 0.1 mm Hg/L/min, or 100 dyne•sec•cm −5 . This value is approximately one tenth the value of systemic vascular resistance.
The use of Equation 1 (from Ohm’s law) is complicated by the fact that resistance is not independent of P pa or P la . As an example, if both P pa and P la were elevated to such a degree that the pressure difference remained unchanged, PVR would nevertheless decrease because of distention of vessels by higher intravascular pressures. Thus inferences regarding resistance changes in the vasculature require consideration of multiple mechanical factors that affect vascular resistance, including not only vascular pressures but also lung volume and inflation pressure. PVR is a function of lung volume because inflation distends some vessels and compresses others, as described later in the discussion of alveolar and extra-alveolar vessels.
PVR can also be potentially modeled by Poiseuille’s law, which, for laminar flow, describes the relationship of resistance (R) of a tube to the tube’s physical characteristics and viscosity of perfusing fluid:
R = 8 π • l r 4 • η
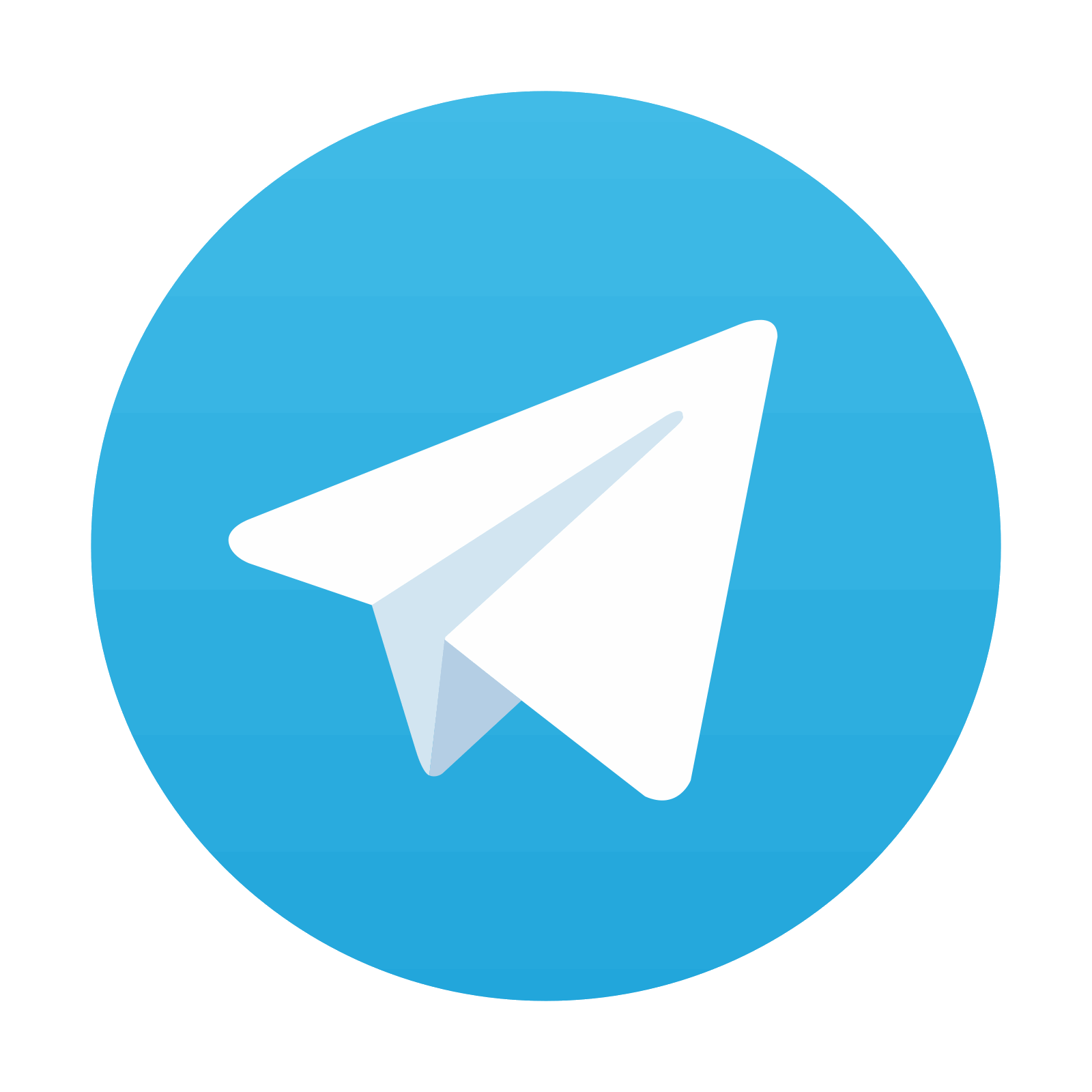
Stay updated, free articles. Join our Telegram channel
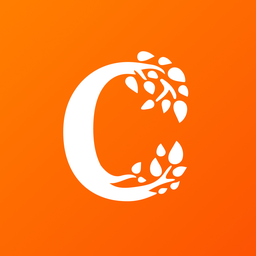
Full access? Get Clinical Tree
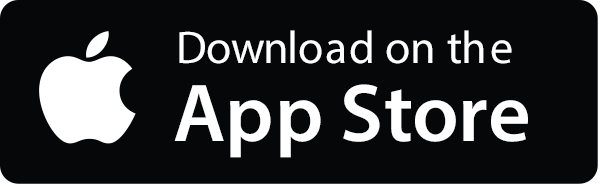
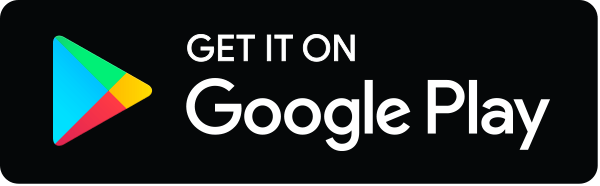