Prosthetic Valves: Introduction
- William A. Zoghbi, MD, MACC
Valvular disease remains prevalent around the world. Despite advancement in valve repair techniques, valve replacement is still common in the adult patient. Although the physical examination can alert the clinician to the presence of prosthetic valve dysfunction, imaging techniques with hemodynamic assessment are often needed to evaluate the function of the prosthesis. Echocardiography with Doppler is currently the method of choice for the noninvasive assessment of prosthetic valves. In 2009, the American Society of Echocardiography published the first guidelines for echocardiographic and Doppler evaluation of prosthetic valve function, which were endorsed by the American College of Cardiology, the American Heart Association, the European Association of Echocardiography, and several other organizations. This introductory chapter focuses on the general principles for a comprehensive evaluation of prosthetic valve function, irrespective of implantation site. Evaluation of individual replacement valves are further detailed in the guidelines and in subsequent chapters.
General overview and approach
Over the past 5 decades, a large variety of prosthetic valves have been developed with the aim of improving valve hemodynamics, increasing durability, and reducing complications. Nevertheless, there is no ideal valve, and all prosthetic valves are prone to dysfunction. The valve types now implanted include, among others, bileaflet and tilting disc mechanical valves, stented porcine and pericardial xenografts, stentless porcine xenografts, and cadaveric homografts. Figures 126.1 and 126.2 show examples of these valves and their echocardiographic images. The guidelines outline the importance of a comprehensive evaluation for optimal assessment of prosthetic valve function. This includes pertinent clinical information, the clinical indication of the examination, symptoms, blood pressure, heart rate, height, weight, and derived body surface area (BSA) ( Table 126.1 ). Height, weight, and BSA are invaluable in diagnosing prosthesis-patient mismatch, although with the higher prevalence of obesity, mismatch may be overestimated by using BSA alone because body mass index may be increased without necessarily a commensurate increase in cardiac output. The date of valve replacement and importantly the type and size of the prosthetic valve are essential, as the latter inherently affect the basic hemodynamics of the valve. With the availability of electronic health records and echocardiographic reporting, this information is more readily available and can be stored for subsequent examinations.


Parameters | |
---|---|
Clinical information | Date of valve replacement |
Type and size of the prosthetic valve | |
Height/weight/body surface area | |
Symptoms and related clinical findings | |
Blood pressure and heart rate | |
Imaging of the valve | Motion of leaflets or occluder |
Presence of calcification on the leaflets or abnormal echo density(ies) on the various components of the prosthesis | |
Valve sewing ring integrity and motion | |
Doppler echocardiography of the valve | Contour of the jet velocity signal |
Peak velocity and gradient | |
Mean pressure gradient | |
Velocity-time integral of the jet | |
Doppler velocity index | |
Pressure half-time in MV and TV. | |
Effective orifice area * | |
Presence, location, and severity of regurgitation † | |
Previous postoperative study(ies), when available | Comparison of listed parameters is particularly helpful in suspected prosthetic valvular dysfunction |
* Effective orifice area using the continuity equation; needs to be compared to normal Doppler values of the valve type and size.
† Transthoracic Doppler is less sensitive to detection of valvular regurgitation in mitral and tricuspid prostheses; transesophageal echocardiography is frequently needed for a more definitive assessment.
Imaging of the valve with transthoracic echocardiography (TTE) from multiple views is recommended with particular attention to the motion of the leaflets or occluder, along with a comprehensive Doppler evaluation of velocity, gradient, and derivation of effective orifice area, where applicable. It is important to note that the TTE/Doppler examination in general should be the first line for evaluation, as it is usually more informative than transesophageal echocardiography (TEE). TTE/Doppler provides comprehensive valve hemodynamics (velocity, gradients, effective area) with more windows for flow interrogation to avoid angulation errors, in addition to assessment of biventricular function and pulmonary pressures. TEE is performed in selected cases for evaluation of suspected structural abnormalities (disc motion, thrombus formation), other complications, and evaluation of the severity and particularly the mechanism of regurgitation ( Box 126.1 ). With the advent of real-time three-dimensional (3D) TEE, the whole prosthetic valve can be seen in real time for the first time with echocardiography ( Fig. 126.3 ). This helps assess motion of the valve and associated complications such as thrombus formation and the extent of perivalvular leaks. Visualization of occluder motion by TEE is best in mitral prostheses and less in aortic and other prostheses. Last, because most prosthetic valves are inherently mildly stenotic and velocities and gradients differ significantly among valves, the acquisition of a baseline Doppler echocardiographic study is strongly recommended early after surgery, for comparison in the serial assessment of valve function. Although the baseline study is ideally performed 2 to 3 months after surgery (when hemodynamics are more stable), it is frequently done before hospital discharge, as other concomitant information is important in patient management, such as ventricular function and whether a pericardial effusion is present.
Valvular regurgitation: Severity and mechanism (mitral and tricuspid valves > aortic valves)
Suspected valve obstruction: Assessment of valve motion, thrombus versus pannus
Evaluation of associated structural abnormalities: Vegetations, thrombi, ring abscess, pseudoaneurysm, fistulas
Atrial, atrial appendage thrombi
Inadequate transthoracic echocardiographic study

General considerations for evaluation of prosthetic valve function
A variable amount of normal or “physiologic” regurgitation is visualized by Doppler echocardiography in all types of mechanical valves, whereas biological valves have minimal or no intrinsic regurgitation at implantation. Tilting-disc and bileaflet valves have built-in leakage when the valve is fully closed that can have different patterns (see Fig. 126.1 ). In general, the same methods used for evaluation of native valvular regurgitation are also applied for prosthetic valve regurgitation. However, two issues are important: First, physiologic transvalvular regurgitation must be recognized and differentiated from pathologic regurgitation. Second, the ability of TTE to detect regurgitation depends on the position of the valve ( Fig. 126.4 ). Imaging of mitral or tricuspid prostheses results in acoustic shadowing in the region behind the valve (respective atrium), which compromises image quality as well as Doppler evaluation. In these cases, TEE is frequently needed to assess the severity of regurgitation and associated structural abnormalities ( Fig. 126.5 ). Clues from the TTE studies for significant mitral regurgitation include high antegrade flow through the prosthesis (high peak E velocity and DVI ratio—see later discussion), low systemic output in comparison with the total output of the left ventricle, and the presence of significant pulmonary hypertension.


Normal prosthetic valves are inherently mildly stenotic compared with native valves. Determinants of gradients include valve type and valve size, as well as flow. This explains in part the wide range of normal parameters of prosthetic valve function in the literature. In general, gradients across prosthetic valves estimated with Doppler by the modified Bernoulli equation (Pressure gradient = 4 V 2 ) correlate well with catheter-derived gradients, provided that angulation between flow and Doppler is minimal (< 20 degrees). Overestimation of gradients with Doppler may occur because of valve design and pressure recovery, particularly in bileaflet valves, where a localized gradient is generated at the smaller orifice between the two leaflets. This is usually more pronounced in small valves and in high-flow states. Although overestimation of gradients by Doppler in these valves results in lower derived effective valve areas compared with the hydraulic formula, it is already accounted for in the published normal values by Doppler echocardiography to which valves are compared.
Another important quantitative parameter in assessing prosthetic valve function is the effective orifice area (EOA, in cm 2 ) of the prosthesis, derived by the continuity equation. EOA complements gradient calculation and offers the advantage of being less dependent on flow. This is calculated as: EOA = Stroke volume/VTI PrV , where VTI PrV is the velocity time integral through the prosthetic valve determined by continuous wave Doppler. Stroke volume is usually derived as cross-sectional area just proximal to the prosthesis (in aortic or pulmonic valves) multiplied by the velocity time integral (VTI) of flow by pulsed Doppler at that site. Stroke volume can also be quantitated from left ventricular volumes derived by 2D or 3D echocardiography. In prosthetic mitral valves, stroke volume calculated at the aortic annulus or pulmonic annulus may be used, provided no significant regurgitation exists.
A simplification of the continuity equation for use in both aortic and mitral prostheses is the Doppler velocity index (DVI), a comparison of VTI PrV to VTI in the LV outflow tract (VTI LVOT ). For prosthetic aortic valves, it is derived as VTI LVOT /VTI PrV and is a good screener for prosthetic valve obstruction. This index does not rely on measurement of the LV outflow tract and is much less dependent on valve size because of the linear relationship of implanted valve size to the size of the left ventricular outflow tract. DVI as calculated for aortic valves is always less than unity because velocity will always accelerate through the prosthesis; it is normally greater than 0.25.
For prosthetic mitral valves, DVI is proposed as the inverse of that in the aortic position : VTI PrV /VTI LVOT . It is normally less than 2.2 for mechanical prostheses. A DVI ratio above 2.2 raises the suspicion of either prosthetic mitral regurgitation or stenosis. , The reason is that VTI PrV is increased in either regurgitation (high flow) or stenosis (obstruction), and VTI LVOT is reduced in mitral regurgitation.
Complications of prosthetic valves
Valvular dysfunction early after surgery is usually related to technical challenges during surgery or early infection. Paravalvular leak is more frequent after debridement of calcium, after repeat valvular surgery, and in older patients. The incidence and nature of late valve dysfunction varies more with the type of prosthesis used, its durability, and its thrombogenicity, as well as patient factors such as the risk of endocarditis. Thromboembolism is determined by the type of heart valve as well as by patient-related factors. Mechanical valves are associated with a higher incidence of thromboembolic complications, although critical valve thrombosis is uncommon. The cause is usually inadequate anticoagulation. Both mechanical and tissue valves are also at risk of interaction between the prosthesis and host to create pannus, which can lead to progressive obstruction. Valve degeneration leading to stenosis and/or regurgitation remains the most frequent complication of biologic valves despite advances in valve design. Echocardiography, particularly TEE, offers a powerful tool to assess these various complications. In patients with valve thrombosis, TEE can also assess the size of the thrombus, which helps in the decision to reoperate or use thrombolysis. , ,
Imaging in the catheterization laboratory: prosthetic valve implantation and repair
Over the past decade, and with the advent of catheter-based interventions on valvular heart disease, imaging with echocardiography has become an integral part of these procedures. The echocardiographer is an essential member of the interventional heart team. The evolving field of “interventional imaging” is poised to be central in structural heart disease. This has included procedures such as transcatheter aortic valve replacement (TAVR), the use of mitral clip for patients with mitral regurgitation, and occluder devices for repair of paraprosthetic valvular leaks. In these conditions, TEE has been essential for optimal guiding of the procedure, particularly mitral clip and occluder devices. Assessment of residual aortic regurgitation during TAVR procedures remains problematic, with the variability of the residual defect extent and number, and is the subject of extensive investigations.
Summary
Echocardiography with Doppler is currently the modality of choice for evaluation and management of prosthetic heart valves as well as native cardiac valves. In general, evaluation of prosthetic valve function is more challenging, based on the variability of inherent mild obstruction observed with the wide range of prosthetic valve types and sizes. Thus, the cardiac history plays a major role in the echocardiographic evaluation by documenting the type and size of the inserted valve. Serial comparison with a baseline postoperative study is also essential in facilitating accurate assessment of valve function. Recent advances in real-time 3D imaging, particularly from the transesophageal approach, offer an important additional dimension in the echocardiographic evaluation of prosthetic valve function. 3D provides a powerful tool to image, for the first time with ultrasound, the motion of the entire valve apparatus and its annulus. This is essential in visualizing motion of the valve and in guiding interventional procedures.
Classification of Prosthetic Valve Types and Fluid Dynamics
- Haïfa Mahjoub, MD
- Jean G. Dumesnil, MD
- Philippe Pibarot, DVM, PhD
- Jean G. Dumesnil, MD
Approximately 290,000 patients required valve replacement in the world in 2003, and this number is estimated to triple to more than 850,000 by 2050. The ideal valve substitute should mimic the characteristics of a normal native valve with excellent hemodynamics, long durability, high thromboresistance, and excellent implantability. Unfortunately, this ideal valve substitute does not exist, and each of the currently available prosthetic valves has inherent limitations. Despite the marked improvements in prosthetic valve designs and procedures over the past decades, valve replacement does not provide a definitive cure to the patient, and the prognosis may be affected by prosthesis-related complications. However, many of these complications can be prevented or their impact minimized through optimal prosthesis selection in the individual patient and careful medical management and follow-up after implantation. In this regard, Doppler echocardiography is the method of choice for the evaluation and follow-up of prosthetic valve function. This evaluation follows the same basic principles used for the evaluation of native valves, with some important particularities and caveats due to significant differences between fluid dynamics of prosthetic valves and those of native valves.
Different types of prosthetic valves
Valve replacement surgery is the standard therapy for severe nonrepairable valvular heart disease. Among the surgical prosthetic valves, there are two main categories: mechanical valves and tissue valves. The stented bioprosthetic valves are by far the most frequently used tissue valves, followed by stentless bioprostheses, homografts, and autografts. During the past decade, there has been a dramatic shift toward use of bioprosthetic valves rather than mechanical valves for surgical aortic valve replacement in the United States. Also in the past decade, transcatheter aortic valve implantation (TAVI) has emerged as a valid alternative to surgical valve replacement in high-risk patients with severe aortic stenosis. At present, more than 1,000 transcatheter bioprosthetic valves have been implanted in the aortic position worldwide.
Surgical prosthetic valves
Mechanical Valves
The three basic types of mechanical valves are ball-cage, tilting-disc, and bileaflet valves. There are major differences in the design of these three types of valves, and their flow pattern and hemodynamics differ markedly. Furthermore, all types of mechanical valves have a normal regurgitant volume that includes a backflow related to the backward motion of the occluder(s) (i.e., the closing volume) and leakage backflow through the components of the prosthesis (leakage volume). This “built-in” regurgitation theoretically prevents blood stasis and thrombus formation by a washing effect. In contrast to pathologic regurgitant jets, normal regurgitant jets are short in duration, narrow, and symmetrical.
Ball-Cage Valves
The only ball-cage valve that is still encountered in patients is the Starr-Edwards 1260, which consists of a Silastic ball with a circular sewing ring and a cage formed by three metal arches located at 120-degree intervals around the sewing ring (see Fig. 126.1 , C ). Antegrade blood flows around the ball, and a large wake is generated in the central part ( Fig. 127.1 , A ). Large regions of flow separation make the valves thrombogenic, and the obstruction to flow caused by the valve results in high pressure gradients. The normal regurgitant flow is composed mainly of the closing volume (2 to 6 mL per beat) that can be visualized on color flow Doppler imaging. This type of valve is no longer implanted, but because of its relatively good durability, several thousand patients still have ball-cage valves.

Monoleaflet Valves
Monoleaflet or tilting-disc valves use a single circular disc that rotates within a rigid annulus to occlude or open the valve orifice. The disc is secured by lateral or central metal struts. The opening angle of the disc relative to the valve annulus ranges from 60 to 80 degrees, resulting in two orifices of different size (see Fig. 126.1 , B ). The jet through the major orifice is semicircular in cross-section, and flow through the minor orifice consists of one, two, or three jets, depending on the number of struts. Minor orifice jet velocities are 30% to 40% lower than those of the major orifice (see Fig. 127.1 , B ). The nonperpendicular opening angle of the valve occluder tends to slightly increase resistance to blood flow, particularly in the major orifices.
To minimize flow turbulence, the preferred orientation of monoleaflet valves in the aortic position is with the major orifice toward the right posterior aortic wall; in the mitral position, the preferred orientation is with the major orifice oriented toward the left ventricular (LV) free wall rather than the septum. Because of the eccentricity of the jet in the major orifice, it is also crucial to use multiwindow interrogation with continuous wave Doppler to get the maximum transvalvular velocity. Normal regurgitant volume is low (5 to 9 mL per beat) and includes the closing volume as well leakage backflow through small gaps around the perimeter of the valve; with the Medtronic Hall valves (Medtronic, Minneapolis, MN) there is a small amount of regurgitation around the central strut (see Fig. 127.1 , B ; Videos 127.1 and 127.2).
Bileaflet Valves
These valves consist of two pyrolytic carbon semicircular leaflets attached by small hinges to a rigid valve ring. The opening angle of the leaflets relative to the annulus plane ranges from 75 to 90 degrees, with the open valve consisting of three orifices: a smaller, slitlike central orifice between the two open leaflets and two larger semi-circular orifices laterally (see Fig. 126.1 , A , and Fig. 127.1 , C ). The pattern of the antegrade transvalvular flow is thus characterized by three separate jets, and because the central orifice is smaller than the two lateral orifices, higher flow velocities may be recorded within the central orifice (see Fig. 127.1 , A , and Fig. 127.2 ). For a given valve annulus size, the effective orifice areas (EOAs) are generally larger for the bileaflet mechanical valves than for the monoleaflet valves ( Tables 127.1 and 127.2 ). Bileaflet valves typically have a small amount (5 to 10 mL per beat) of normal regurgitation. On Doppler color flow imaging, two converging regurgitant jets originating from the pivot points of the valve discs and a smaller central jet are often seen. Smaller jets around the closure rim of the leaflets may also be appreciated (see Fig. 127.1 , A ; Videos 127.3 and 127.4). When implanted in the aortic position, there is no clear advantage to a specific orientation of the leaflet opening plane relative to the aortic root. For the mitral position, it is suggested that an orientation perpendicular to the normal plane of mitral valve opening may be optimal. Available bileaflet valves include the St. Jude Medical (Saint Paul, MN) and Carbomedics (Sorin Group, Milan, Italy), On-X, (MRCI, Austin, TX) and ATS (Medtronic) valves.

Prosthetic Valve Size (mm) | 19 | 21 | 23 | 25 | 27 | 29 |
---|---|---|---|---|---|---|
Stented Bioprosthetic Valves | ||||||
Mosaic | 1.1 ± 0.2 | 1.2 ± 0.3 | 1.4 ± 0.3 | 1.7 ± 0.4 | 1.8 ± 0.4 | 2.0 ± 0.4 |
Hancock II | — | 1.2 ± 0.2 | 1.3 ± 0.2 | 1.5 ± 0.2 | 1.6 ± 0.2 | 1.6 ± 0.2 |
Carpentier-Edwards Perimount | 1.1 ± 0.3 | 1.3 ± 0.4 | 1.50 ± 0.4 | 1.80 ± 0.4 | 2.1 ± 0.4 | 2.2 ± 0.4 |
Carpentier-Edwards Magna | 1.3 ± 0.3 | 1.5 ± 0.3 | 1.8 ± 0.4 | 2.1 ± 0.5 | — | — |
Biocor (Epic) | 1.0 ± 0.3 | 1.3 ± 0.5 | 1.4 ± 0.5 | 1.9 ± 0.7 | — | — |
Mitroflow | 1.1 ± 0.2 | 1.2 ± 0.3 | 1.4 ± 0.3 | 1.6 ± 0.3 | 1.8 ± 0.3 | — |
Trifecta | 1.41 | 1.63 | 1.81 | 2.02 | 2.30 | 2.35 |
Stentless Bioprosthetic Valves | ||||||
Medtronic Freestyle | 1.2 ± 0.2 | 1.4 ± 0.2 | 1.5 ± 0.3 | 2.0 ± 0.4 | 2.3 ± 0.5 | — |
St. Jude Medical Toronto SPV | — | 1.3 ± 0.3 | 1.5 ± 0.5 | 1.7 ± 0.8 | 2.1 ± 0.7 | 2.7 ± 1.0 |
Prima Edwards | — | 1.3 ± 0.3 | 1.6 ± 0.3 | 1.9 ± 0.4 | — | — |
Mechanical Valves | ||||||
Medtronic-Hall | 1.2 ± 0.2 | 1.3 ± 0.2 | — | — | — | — |
St. Jude Medical Standard | 1.0 ± 0.2 | 1.4 ± 0.2 | 1.5 ± 0.5 | 2.1 ± 0.4 | 2.7 ± 0.6 | 3.2 ± 0.3 |
St. Jude Medical Regent | 1.6 ± 0.4 | 2.0 ± 0.7 | 2.2 ± 0.9 | 2.5 ± 0.9 | 3.6 ± 1.3 | 4.4 ± 0.6 |
MCRI On-X | 1.5 ± 0.2 | 1.7 ± 0.4 | 2.0 ± 0.6 | 2.4 ± 0.8 | 3.2 ± 0.6 | 3.2 ± 0.6 |
Carbomedics Standard and Top Hat | 1.0 ± 0.4 | 1.5 ± 0.3 | 1.7 ± 0.3 | 2.0 ± 0.4 | 2.5 ± 0.4 | 2.6 ± 0.4 |
ATS Medical * | 1.1 ± 0.3 | 1.6 ± 0.4 | 1.8 ± 0.5 | 1.9 ± 0.3 | 2.3 ± 0.8 | — |
* For the ATS medical valve, the label valve sizes are 18, 20, 22, 24, 26 mm.
Prosthetic Valve Size (mm) | 25 | 27 | 29 | 31 | 33 |
---|---|---|---|---|---|
Stented Bioprosthetic Valves | |||||
Medtronic Mosaic | 1.5 ± 0.4 | 1.7 ± 0.5 | 1.9 ± 0.5 | 1.9 ± 0.5 | — |
Hancock II | 1.5 ± 0.4 | 1.8 ± 0.5 | 1.9 ± 0.5 | 2.6 ± 0.5 | 2.6 ± 0.7 |
Carpentier-Edwards Perimount | 1.6 ± 0.4 | 1.8 ± 0.4 | 2.1 ± 0.5 | — | — |
Mechanical Valves | |||||
St. Jude Medical Standard | 1.5 ± 0.3 | 1.7 ± 0.4 | 1.8 ± 0.4 | 2.0 ± 0.5 | 2.0 ± 0.5 |
MCRI On-X * | 2.2 ± 0.9 | 2.2 ± 0.9 | 2.2 ± 0.9 | 2.2 ± 0.9 | 2.2 ± 0.9 |
* The On-X valve has just one size for 27- to 29-mm and 31- to 33-mm prostheses. In addition, the strut and leaflets are identical for all sizes (25- to 33-mm); only the size of the sewing cuff is different.
Tissue Valves
Tissue valves include stentless or stented bioprostheses made of porcine or bovine tissues, homografts from human cadaveric sources, and autografts of pericardial or pulmonary valve origin.
Stented Bioprostheses
The traditional design of a heterograft valve consists of three biologic leaflets made from the porcine aortic valve or bovine pericardium treated with glutaraldehyde to reduce its antigenicity. The leaflets are mounted on a metal or polymeric stented ring; they open to a circular orifice in systole, resembling the anatomy of the native aortic valve (see Fig. 126.2 , A ). The vast majority of bioprosthetic valves are treated with anticalcification agents or processes. A circular central flow field with a relatively flat flow velocity profile characterizes the normal flow pattern in stented bioprostheses (see Fig. 127.1 , D , and Fig. 127.2 , A ). in vitro studies show that flow stagnation occurs on the outflow surfaces of the bioprosthetic leaflets during systole (see Fig. 127.1 , D ). Although bioprosthetic valves are considered to be relatively nonthrombogenic, flow turbulence and stagnation that are confined to the perimeter of flow stream may increase the likelihood of thromboembolic events.
For a given valve annulus size, the EOAs are generally smaller for stented bioprostheses than for bileaflet mechanical valves (see Tables 127.1 and 127.2 ). However, the hemodynamic performance of stented bioprosthetic valves has improved substantially with newer valve generations.
A small degree of central regurgitation (< 1 mL) is also often observed in bioprosthetic valves, and more frequently in bovine pericardial valves (see Fig. 127.1 , D ; Videos 127.5 and 127.6). The available stented bioprostheses include Carpentier-Edwards Perimount, Magna, and S.A.V. valves (Edwards Lifesciences, Irvine, CA), Epic (St. Jude Medical), Biocor (St. Jude Medical), Hancock II (Medtronic), Mitroflow (Sorin Group), Mosaic (Medtronic), and Trifecta (St. Jude Medical).
Stentless Bioprostheses
In an effort to improve valve hemodynamics and durability while retaining the advantages of a bioprosthetic valve, several types of stentless bioprosthetic valves have been developed. Stentless bioprostheses are manufactured from intact porcine aortic valves or from bovine pericardium (see Fig. 126.2 , B ). Stentless bioprosthetic valves have been used only in the aortic position; they may be implanted in the subcoronary position or as an inclusion cylinder, a miniroot, or a total root replacement. The most frequently used techniques are the subcoronary and the total root. The advantage of a subcoronary implantation is to avoid the reimplantation of the coronary arteries; on the other hand, there is a risk of central valvular insufficiency in the late postoperative course due to continued dilation of the aortic root. The first generation of stentless bioprostheses include the Medtronic Freestyle, the Toronto SPV (St. Jude Medical), and the Prima-Edwards (Edwards Lifesciences). New generations of stentless valves (Shelhigh Super Stentless aortic valve [Shelhigh Inc., Union, NJ], Sorin Pericarbon Freedom valve [Sorin Group] are easier to implant because they require only one layer of suture.
The echocardiographic appearance mimics a normal aortic valve, with increased echogenicity in the annulus and the aortic root from the surgical procedure and the layer of fabric supporting the graft (Videos 127.7 and 127.8). The flow accelerates uniformly through the valve, and downstream the flow has a relatively flat profile. The EOAs are generally larger and gradients lower in stentless valves than in stented valves (see Table 127.1 ). The hemodynamic performance of the stentless valves often improves within the first 3 to 6 months of follow-up because of remodeling of the aortic root.
Sutureless Bioprostheses
The sutureless stent-mounted bioprosthetic valves are designed to replace a diseased native or malfunctioning prosthetic aortic valve via open heart surgery with sutureless positioning and anchoring at the implantation site ( Fig. 127.3 , A; Videos 127.9 and 127.10). These valves can be implanted with minimally invasive cardiac surgery through either a partial sternotomy or a right mini-thoracotomy. This type of valve allows substantial reduction of aortic cross-clamp time. The sutureless valves include the Perceval (Sorin Group), the 3F Enable valve (Medtronic), and the Intuity (Edwards Lifesciences).

Aortic Homografts
Aortic valve homografts are harvested from human cadavers within 24 hours of death as blocks of tissue comprising the ascending aorta, aortic valve, a portion of interventricular septum, and the anterior mitral valve leaflet (see Fig. 127.3 , B ). They are treated with antibiotics and cryopreserved at − 196 degrees. They are now most commonly implanted in the form of a total root replacement with reimplantation of the coronary arteries. Homografts have excellent hemodynamics with antegrade velocities similar to those of a native valve. Regurgitation is rare with the root replacement technique. However, with subcoronary implantation, nearly 25% of patients develop moderate/severe aortic regurgitation by 2 years and up to 5% require reoperation for significant aortic regurgitation. Long-term durability beyond 10 years is not superior to that of current-generation pericardial bioprostheses.
Pulmonary Autografts (Ross Procedure)
The native pulmonary valve is harvested as a small cylinder consisting of the pulmonary valve, annulus, and proximal main pulmonary artery (see Fig. 127.3 , C ). Autografts are most often implanted as complete root replacement with reimplantation of the coronaries. The pulmonary valve and right ventricular outflow tract are then replaced with a pulmonary homograft. The procedure requires two separate valve operations and a longer cardiopulmonary bypass time and has a steep learning curve. The hemodynamics of the pulmonary autografts are similar to those of a normal native aortic valve both at rest and with exercise. However, the pulmonary homograft is subject to early degeneration and failure. Autografts have the ability to increase in size during childhood growth and are usually reserved for children and young adults. They are also nonthrombogenic and resistant to infections. However, the autograft may fail in some patients because of dilation of the autograft and ensuing aortic regurgitation.
Transcatheter bioprosthetic valves
Transcatheter aortic valve implantation is emerging as an alternative to surgical aortic valve replacement in patients with symptomatic aortic stenosis who are considered to be at high or prohibitive operative risk. Two main types of transcatheter aortic valves are currently used: balloon-expandable valves and self-expanding valves.
Balloon-Expandable Valves
The first two generations of the Edwards balloon-expandable valves comprised three leaflets fabricated with equine pericardium (Cribier-Edwards, Edwards Lifesciences) or bovine pericardium (Edwards SAPIEN) mounted in a stainless steel frame, available in two sizes, 23 and 26 mm. The valves were implanted using 22 French and 24 French delivery catheters. The Edwards SAPIEN XT (Edwards Lifesciences) is the third generation and consists of a three-leaflet pericardial bovine valve mounted in a cobalt chromium frame. It is available in 20-, 23-, 26-, and 29-mm sizes and is implanted using 18 French, 19 French, and 22 French delivery catheters (see Fig. 126.2 , C , and Fig. 127.3 , D, and Video 127.11). The transfemoral retrograde approach is most often used today and consists of entering through the femoral artery and backtracking through the aorta to the aortic valve. An accurate evaluation of the iliofemoral anatomy helps to determine the appropriateness of the transfemoral approach for each patient. If the iliofemoral arteries are too small, diseased, and/or tortuous, a transapical or transaortic approach may be used. The transapical approach requires a small left lateral thoracotomy and a direct puncture of the left ventricular apex and thus leads to a greater degree of myocardial injury. A transaortic approach is also possible.
The normal EOAs of the SAPIEN valves range between 1.3 and 2.0 cm 2 . , As opposed to surgical prostheses, the EOA of a given prosthesis size varies significantly depending on the size of patient’s aortic annulus. Several studies, including the randomized trial PARTNER-I Cohort A, have demonstrated that, for a given aortic annulus size, balloon-expandable valves have larger EOAs and lower gradients compared to surgical bioprosthetic valves. , On the other hand, unlike surgical bioprosthetic valves, paravalvular regurgitation is common following transcatheter aortic valve implantation. Mild to moderate regurgitation occurs in 30% to 80% of cases, with 5% to 20% being moderate to severe. , , Moderate to severe paravalvular regurgitation is associated with a twofold increase in mortality. Paravalvular leak can also cause high blood shear stress and thereby damage to blood cells and coagulation factors, which could increase the risk of hemolysis, thromboembolic events, and/or bleeding. The most recent generation of balloon-expandable valves includes a skirt to reduce paravalvular regurgitation.
Self-expanding Valves
The first generation of the CoreValve system consisted of a self-expanding nitinol frame with a bovine pericardial heart valve and was implanted using a 25 French delivery catheter (see Fig. 127.3 , E ). The second generation of the CoreValve system consisted of three leaflets of porcine pericardium seated higher in the nitinol frame to provide true supra-annular placement and was implanted using a 21 French delivery catheter. The third generation differs slightly from the previous one in the sealing skirt to reduce paravalvular regurgitation, available in 26-, 29-, and 31-mm sizes and implanted using an 18 French delivery catheter. The CoreValve (Medtronic) is mostly implanted using the transfemoral approach. However, the subclavian and transaxillary approaches can also be used if the transfemoral access is not feasible. Some studies suggest that the CoreValve has slightly larger EOAs and lower gradients than the SAPIEN valves but higher incidence of paravalvular regurgitation.
The flow pattern of transcatheter valves differs from that of surgical bioprostheses in the sense that there are two levels of flow acceleration at the inflow aspect of the valve: a first level when the flow enters the apical portion of the stent, and a second level when the flow passes through the prosthetic valve leaflets ( Fig. 127.4 ). For that reason, it is generally recommended to measure the left ventricular outflow tract (LVOT) diameter and velocity immediately proximal to the apical end of the stent when measuring the stroke volume and EOA of transcatheter valves. , However, if the stent sits low in the LVOT, which may occur more frequently with the self-expandable prostheses, it may be preferable to measure the LVOT diameter and velocity within the proximal portion of the stent below the bioprosthetic leaflets.

Pressure recovery
Doppler-echocardiography is the method of choice for the evaluation of prosthetic valve function. However, several studies have reported that Doppler may overestimate the gradient across native, mechanical, or bioprosthetic aortic valves when compared with catheter measurements. , As blood flow velocity decelerates between the aortic valve and the ascending aorta, part of the kinetic energy is reconverted back to static energy (i.e., pressure) because of a phenomenon called pressure recovery, and hence the net gradient between the left ventricle and the ascending aorta (i.e., the gradient measured by catheter) is less than the maximum pressure gradient measured by Doppler at the level of the vena contracta (see Fig. 127.2 ). The extent of pressure recovery is determined by the ratio between the valve EOA and the cross-sectional area of the downstream chamber, that is, the ascending aorta in the case of native or prosthetic aortic valves. Hence, pressure recovery generally becomes clinically relevant in patients with smaller aortas, that is, those with an aorta diameter of 30 mm or less at the sinotubular junction. , In these patients, it is thus appropriate to account for pressure recovery by using the simple formula proposed by Garcia and colleagues to calculate the energy loss coefficient: <SPAN role=presentation tabIndex=0 id=MathJax-Element-1-Frame class=MathJax style="POSITION: relative" data-mathml='ELC=EOA×AA/AA−EOA’>ELC=(EOA×AA)/(AA−EOA)ELC=EOA×AA/AA−EOA
ELC = EOA × A A / A A − EOA
, where A A is the cross-sectional area of the aorta measured at about 1 cm downstream of the sinotubular junction. The energy loss coefficient should be indexed for body surface area (i.e., energy loss index) to account for the change in cardiac output related to body size. An energy loss index less than 0.55 to 0.6 cm 2 /m 2 is suggestive of a significant prosthetic valve stenosis or patient-prosthesis mismatch (PPM). Not accounting for pressure recovery in patients with small aortas (i.e., < 30 mm) may cause overestimation of prosthetic valve stenosis and/or PPM by Doppler echocardiography and lead to unwarranted investigations or interventions. The pressure recovery is generally negligible in the case of mitral prostheses because the downstream chamber (i.e., the left ventricle) is large relative to the EOA of the prosthesis.
Localized high gradient in bileaflet mechanical valves
Significant pressure recovery may occur downstream of aortic valves regardless of their type (i.e., native, bioprosthetic, or mechanical). This phenomenon should not, however, be mistaken for the phenomenon of localized high gradient that is specific of bileaflet mechanical valves (see Fig. 127.2 ; Fig. 127.5 ). A localized high gradient may indeed be recorded through the central orifice of bileaflet mechanical valves, and this phenomenon may yield to overestimation of gradient and underestimation of EOA regardless of the position (aortic or mitral) of the prosthesis. Because the central orifice is smaller than the lateral orifices, the blood flow velocity may be locally higher within the inflow aspect of the central orifice, and continuous wave Doppler may record this high velocity (see Figs. 127.2 and 127.5 ). The prevalence, magnitude, and predictors of this phenomenon are not fully understood but are probably related to prosthetic valve size and design (ratio of the size of the central orifice to that of lateral orifices) and flow conditions. , Also, given that the localized high-velocity region is very small and located at the inflow of the central orifice (see Fig. 127.5 ), the recording of this velocity is highly inconsistent and may vary from one patient to the next and even from one visit to the next in a given patient, depending on the direction and angulation of the Doppler beam.

Prosthesis-patient mismatch
Prosthesis-patient mismatch occurs when the EOA of a normally functioning prosthesis is too small in relation to the patient’s body size (and thus cardiac output requirements), resulting in abnormally high postoperative gradients. Hence, PPM is not an intrinsic prosthesis dysfunction per se. The most widely accepted and validated parameter for identifying PPM is the indexed EOA, that is, the EOA of the prosthesis divided by the patient’s body surface area. Table 127.3 shows the cutoff values of indexed EOA generally used to identify PPM and quantify its severity. Moderate PPM may be quite frequent both in the aortic (20% to 70%) and mitral (30% to 70%) positions, whereas the prevalence of severe PPM ranges from 2% to 10% in both positions. , PPM is associated with worse hemodynamics; less regression of left ventricular hypertrophy and pulmonary hypertension; worse functional class, exercise capacity, and quality of life; more cardiac events; and lower survival. PPM can largely be prevented by using a prospective strategy at the time of operation consisting of systematically calculating the “projected” indexed EOA of the prosthesis to be inserted (projected indexed EOA is derived from the normal reference values of EOA provided in the literature for the different types and sizes of prostheses; see Tables 127.1 and 127.2 ) and, in the case of anticipated PPM, to consider alternative procedures such as insertion of a better-performing valve substitute (e.g., the newer generation of supra-annular bioprostheses or mechanical valves, stentless bioprostheses, homografts, or transcatheter valves) or insertion of a larger prosthesis by mean of aortic root enlargement. The prevention of PPM in the mitral position represents a much greater challenge than in the aortic position. Indeed, mitral valve surgery does not allow annular enlargement, and the implantation of a homograft or a stentless prosthesis is technically more demanding and associated with poor long-term durability. Hence, the only alternative at present is the implantation of a prosthesis having a larger EOA for a given annulus size, which unfortunately may not be sufficient to completely avoid PPM in some cases.
Mild or Not Clinically Significant | Moderate | Severe | |
---|---|---|---|
Aortic position | > 0.85 (0.8-0.9) | ≤ 0.85 (0.8-0.9) | ≤ 0.65 (0.6-0.7) |
Mitral position | > 1.2 (1.2-1.3) | ≤ 1.2 (1.2-1.3) | ≤ 0.9 (0.9) |
Numbers in parentheses represent the range of threshold values that have been used in the literature. |
Conclusion
Several prosthetic valve designs are currently available for valve replacement procedures, and many new models will be introduced in the future owing to the rapidly growing expansion of transcatheter valve therapy. Optimal Doppler-echocardiographic evaluation of prosthesis structure and function requires a good knowledge of the specifics of each type of prosthesis in terms of valve design, implantation technique, and fluid dynamics.
Aortic Prosthetic Valves
- Damian Roper, MBChB
- Darryl J. Burstow, MBBS
Transthoracic echocardiography (TTE) is established as the reference imaging modality for the routine evaluation of prosthetic valves because of the comprehensive anatomic, functional, and hemodynamic data it provides combined with its excellent safety profile. However, the unique acoustic properties of prosthetic valves can impair the echocardiographic examination, resulting in reduced diagnostic accuracy. To reduce errors in interpretation, an understanding of the distinctive structural and hemodynamic features of prosthetic valves is required combined with a standardized TTE examination protocol that includes integration of all data obtained from two-dimensional, spectral, and color flow Doppler modalities. In addition, knowledge of the limitations of TTE will enable the appropriate selection of cases where additional imaging procedures, such as transesophageal echocardiography (TEE) and computed tomography (CT) or cine-fluoroscopy, may be required.
Standard transthoracic echocardiographic assessment of aortic prosthetic valve function
Two-Dimensional Imaging
Before imaging, the type and size of the prosthetic valve and the date of insertion should be established. The appearance and motion of the leaflets/occluder can then be assessed. Careful attention to specific imaging planes will reduce the problems caused by acoustic shadowing and improve diagnostic yield. If the ultrasound beam can be oriented parallel to the direction of occluder opening, acoustic shadowing across the plane of the valve is reduced with resultant improvement in visualization of occluder motion. This is particularly relevant in the assessment of aortic prostheses where the apical five-chamber view can provide an improved view of the leaflets/occluder.
Doppler Parameters Used to Assess Aortic Prosthetic Valve Function
All prosthetic valve designs cause variable degrees of obstruction to flow resulting in measureable gradients. Doppler assessment allows the noninvasive measurement of flow velocities across prosthetic valves from which gradients are calculated. As with native aortic valve stenosis, Doppler interrogation of the aortic prosthesis should be performed from multiple acoustic windows to ensure that the highest peak velocity and derived gradients have been obtained. Importantly, valve gradients are determined by both volumetric flow and prosthetic valve area. Thus, in addition to valve gradients, the derivation of flow-independent parameters of prosthetic valve function such as effective orifice area (EOA) and Doppler velocity index (DVI) is essential ( Box 128.1 ).
AVR velocity (CW Doppler)
- •
Peak velocity (V 2 ) cm/sec
- •
Maximum gradient (4 <SPAN role=presentation tabIndex=0 id=MathJax-Element-2-Frame class=MathJax style="POSITION: relative" data-mathml='V22′>V22V22
V 2 2
) mm Hg
- •
Mean gradient mm Hg
- •
AVR VTI cm
LVOT velocity (PW Doppler)
- •
Peak velocity (V 1 ) cm/sec
- •
LVOT VTI cm
LVOTd cm
<SPAN role=presentation tabIndex=0 id=MathJax-Element-3-Frame class=MathJax style="POSITION: relative" data-mathml='DVI=V1÷V2′>DVI=V1÷V2DVI=V1÷V2
DVI = V 1 ÷ V 2
<SPAN role=presentation tabIndex=0 id=MathJax-Element-4-Frame class=MathJax style="POSITION: relative" data-mathml='EOAcm2=LVOTVTI×LVOTd2×0.785/AVRVTI=LVOTVTI×LVOTCSA/AVRVTI’>EOA(cm2)=(LVOTVTI×(LVOTd2×0.785)/AVRVTI)=(LVOTVTI×LVOTCSA/AVRVTI)EOAcm2=LVOTVTI×LVOTd2×0.785/AVRVTI=LVOTVTI×LVOTCSA/AVRVTI
EOA cm 2 = LVOT VTI × LVOTd 2 × 0.785 / AVR VTI = LVOT VTI × LVOT CSA / AVR VTI
CSA , Cross-sectional area; CW , continuous wave; DVI , Doppler velocity index; EOA , effective orifice area; LVOT , left ventricular outflow tract; VTI , velocity-time integral.
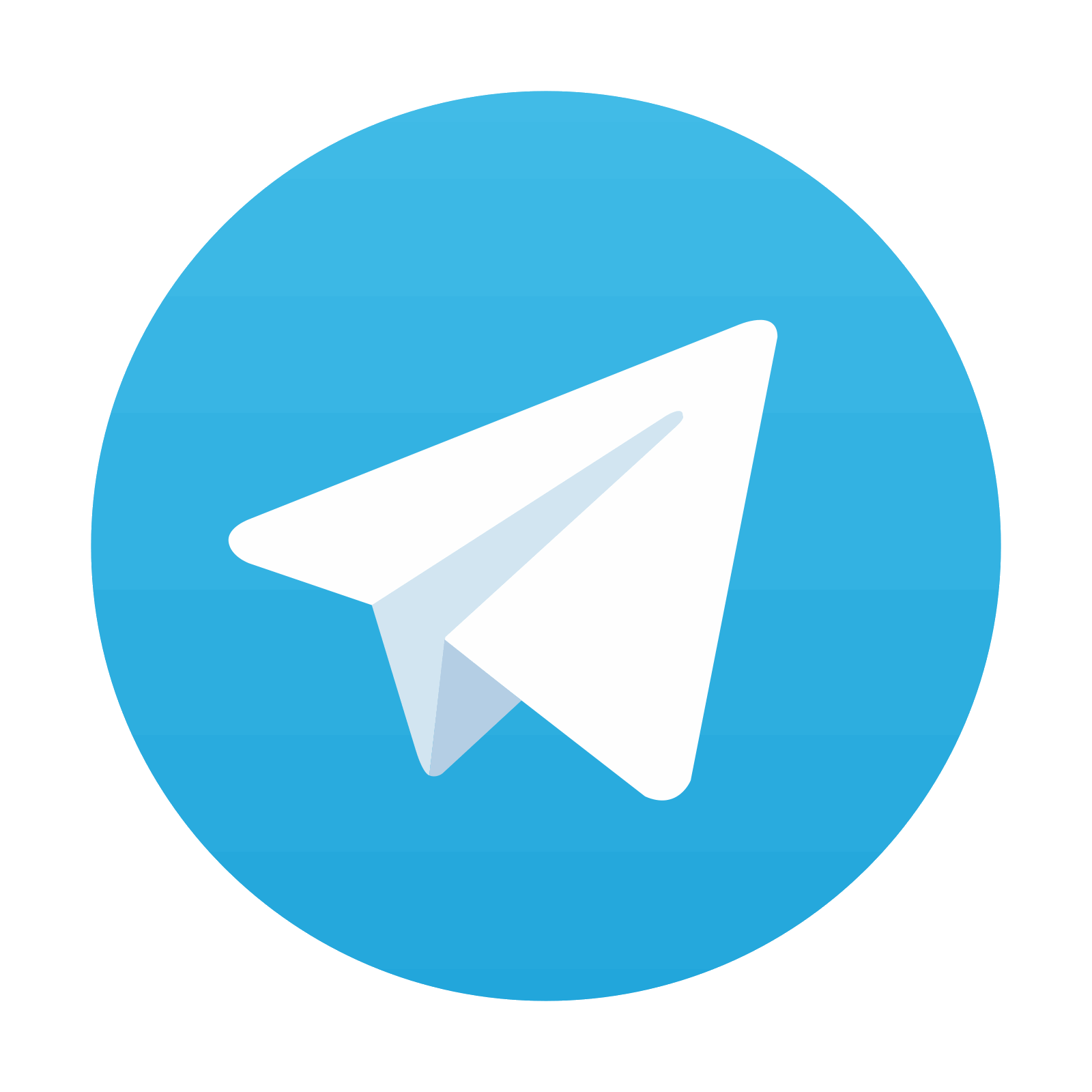
Stay updated, free articles. Join our Telegram channel
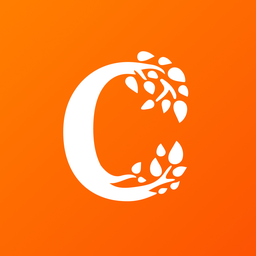
Full access? Get Clinical Tree
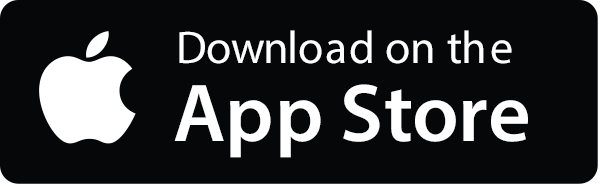
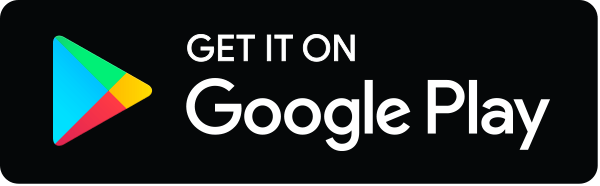
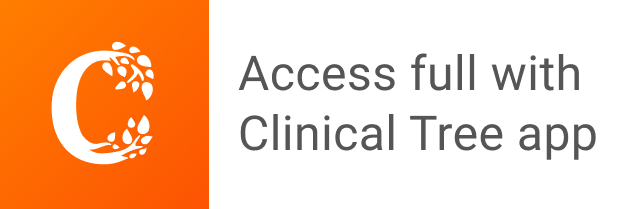