Fig. 4.1
Sequential image generation (Reprinted from Miele FR. Essentials of Ultrasound Physics: the Board Review Book; Miele Enterprises, LLC; 2008: 104. ©2008 Miele Enterprises, LLC. with permission.)
Absorption
To further understand the ultrasound image, it is important to discuss the mechanical interaction between the sound wave and the medium (body) and how these interactions relate to how images are presented. As the sound waves propagate through the tissue, some of the wave energy is lost as heat through absorption. Absorption rates within the tissue types are important for two reasons. First, all of the energy that absorbs results in less signal to reflect back, decreasing sensitivity. Second, absorption leads to tissue heating which can cause metabolic breakdown resulting in tissue damage (referred to as a thermal bioeffect.). It is valuable to know that absorption increases nonlinearly with increasing frequency and depth, implying that higher frequencies are generally not very useful for deeper imaging.
Reflection
Reflectivity is based on the wavelength of the sound wave relative to the structure geometry as well as the heterogeneity of the acoustic properties of the tissues. When structures are large with respect to the wavelength, a very angle-dependent type of reflection occurs, called specular reflection. As the surface appears “rough” with respect to the wavelength, a less angle-dependent type of reflection occurs referred to as backscattering (or sometimes just scattering). When wavelengths are large with respect to the structure, a weak reflective mechanism of Rayleigh scattering occurs. The wavelength is given as:
where c = speed of sound and f = operating (transmit) frequency.

From this equation, we see that shorter wavelengths result from:
Higher operating frequency (chosen by the person scanning but generally restricted by the required imaging depth)
Lower propagation velocities (determined by the properties of the medium)
When the transmitted pulse encounters a large change in the acoustic properties of the tissue (referred to as the acoustic impedance), a large reflection results. Similarly, when only a small change in acoustic impedance exists, a small reflection occurs. The varying reflecting echoes return to the transducer and are converted into electrical signals which are then interpreted by the system and converted to color scale (usually gray scale) to produce an image. Large reflections are generally mapped to brighter shades of white, whereas weaker reflections are presented as various shades of gray. No reflection detected either because of medium homogeneity or inadequate sensitivity is presented as black. When differing tissues have very similar acoustic impedances, the contrast between the tissues will be low, and the possibility exists that the two different tissue types will not be differentiable in the image. Such situations can occur when a mass has acoustic properties similar to the surrounding tissue or in cases of a lipid-rich plaque with a thin cap. The ability to differentiate tissues based on brightness is referred to as contrast resolutio n.
Depth and Speed of Sound
Mapping the returning echoes to a d epth location in the image is a simple matter of applying the distance equation, based on the assumption that sound travels approximately 1540 m/s in soft tissue. The assumed speed of 1540 m/s is equivalent to 6.5 μs (microseconds) per cm of travel or equivalent to 13 μs (microsecond) per cm of imaging depth because of the roundtrip effect (Fig. 4.2). Of course, when the true speed of sound within the body varies from the assumed speed of sound, echoes are mapped either too shallow (higher than the assumed 1540 m/s) or too deep (lower than the assumed 1540 m/s).


Fig. 4.2
Speed of sound in tissue (Reprinted from “Ultrasound Physics & Instrumentation, 5th Edition” by F. R. Miele, p. 182. Copyright 2013 by Miele Enterprises, LLC. Reprinted with permission.)
Spatial Resolution
The ability to resolve ch anges in time (temporal resolution) and the ability to distinguish between tissues based on differences in brightness (contrast resolution) have already been mentioned. The ability to spatially resolve structures within an image is subdivided into three dimensions: axial (depth or longitudinal) resolution, lateral resolution, and elevation resolution. In essence the best spatial resolution is obtained with a short pulse (axial resolution) and a narrow beam (lateral and elevation resolution). Shorter spatial pulse lengths result in better axial resolution with a greater ability to differentiate structures separated along the axis of the beam. Additionally, shorter pulses result in more accurate measurements in the axial direction. This concept is of course important when measurement accuracy matters such as when making intima-media thicknes s (IMT) measurements. Narrower beams result in better lateral and elevation resolution. For conventional 1-D array transducers, the lateral beam dimension can be changed by varying the depth of the focus (or foci when multiple foci are activated). When the focal depth is changed, more or fewer elements of the transducer are activated, and the timing of the transmit pulses varied to each element to achieve a deeper or a shallower focus. However, for 1-dimensional (1-D) arrays, there is only one element in the elevation plane (which corresponds to the “slice thickness” plane), and hence the focus cannot be varied in elevation (fixed elevational focus). In the elevation plane, most 1-D arrays have an acoustic lens built into the surface of the transducer that fixes the focus at a specific “optimal” depth for the application for which the transducer was designed. In general, transducers intended primarily for shallow imaging will have a shallower elevation focus by design since the transducer is not intended for deeper imaging applications.
The ability to differentiate a specific tissue from surrounding tissue is related to the acoustic impedance mismatch between the two tissues. In many cases, the acoustic properties of the two tissues may be only slightly dissimilar, resulting in poor or no differentiation. This problem exists frequently with masses such as liver and kidney lesions and with fresh thrombus which has acoustic properties only slightly different than the surrounding blood. In these cases, even low-level noise can obscure the small contrast that does exist. This problem is exacerbated by the fact that the range of signal amplitudes reflecting from the body well exceeds the range of signals perceptible to the human eye. In many instances the ratio of the largest to smallest signals reflecting from the body (the dynamic range) exceeds 80 dB (10,000 levels), whereas the human eye can perceive simultaneously less than 36 dB (fewer than 64 shades of gray). As a result, the imaging system must compress the signal dynamic range to better map into the dynamic range of the human eye. Of course, the process of compression implies that differences become smaller, potentially mapping small signal differences to the same grayscale appearance.
Video Compression
Ultrasound systems allow the user to change the video compression applied to an image (different systems use different terminology for this control such as gray scale, compression, dynamic range, and post processing). By having multiple compression maps with varying slopes, the expectation is that when one map fails to display certain signals, a different map will succeed. This expectation of course can only be realized if the person scanning actively changes the compression settings to determine if any signals are being missed. The following two images (Fig. 4.3) are identical except for the applied compression map. Notice that the image on the right gives evidence of a thrombus which is not evident in the image on the left.


Fig. 4.3
Compression settings can affect interpretation (Reprinted from Miele FR. Ultrasound Physics & Instrumentation. Miele Enterprises, LLC; 2006: 342. ©2006 Miele Enterprises, LLC. with permission.)
In addition to gray scale (compression maps), there are many system parameters that should be optimized to decrease the chances of not visualizing weak signals from the surrounding tissue.
The list includes:
Using the correct transmit frequency (adequate penetration and best possible resolution)
Using adequate transmit power
Using harmonics (unless too deep)
Using compound imaging
Varying compression maps to improve contrast resolution
Some of the advanced techniques will be discussed in the upcoming section.
Advanced Imaging Technologies
In addition to improvements in electronics and transducers, there are many advanced techniques which have significantly improved the quality of ultrasound images over the last 10 years. An exhaustive treatment of imaging technologies is beyond the scope of this chapter, but two of the most important techniques are covered in the upcoming section.
Harmonic Imaging
The principles of second harmonic imaging are simple. For harmonic imaging the system transmits at a lower frequency, referred to as the fundamental, and then receives and processes signals at twice the fundamental signal, referred to as the second harmonic. Of course the complexity comes in understanding how fundamental signals are converted into harmonic signals and ,more importantly, in understanding the benefits and limitations of harmonic imaging.
There is a nonlinear response of tissue to the compression and rarefaction of sound waves. During compression, the density of the tissue molecules increases, resulting in a slight increase in propagation speed, whereas during rarefaction, the density decreases such that there is a slight decrease in the propagation velocity. The result is that the wave becomes distorted (Fig. 4.4) as it propagates through the tissue, introducing harmonic energy into the wave. The harmonics are not limited to second harmonics, but as of now, ultrasound systems are not processing higher-order harmonics as each higher harmonic signal becomes weaker and are currently too weak for adequate image generation. The generation of harmonic energy is very nonlinear with the beam intensity (related to the parameter called the mechanical index (MI)). In essence, a slightly lower intensity (lower MI) can result in significantly less harmonic signal generation. For this reason, harmonic imaging is very sensitive to where the beam focus is placed as well to the overall transmit power used.


Fig. 4.4
Distortion of wave producing harmonic energy (Reprinted from “Ultrasound Physics & Instrumentation, 5th Edition” by F. R. Miele, p. 328. Copyright 2013 by Miele Enterprises, LLC. Reprinted with permission.)
Making use of the harmonic signals generated by nonlinear propagation requires transducers with enough bandwidth (range of frequencies over which the transducer can operate) so that the transducer can transmit at the fundamental frequency and receive signals at twice the fundamental frequency (the second harmonic signal). Modern transducers capable of such large dynamic range are commonly referred to as ultra-wide bandwidth transducers. When the system processes the returning echoes, a filter is applied to look at just the second harmonic bandwidth, explicitly attempting to not overlap with the transmit bandwidth. Any overlap between the fundamental and harmonic bandwidths allows for the clutter from the fundamental to degrade the image quality of the harmonic signal. In order to reduce the overlap between fundamental and harmonic bandwidth, longer transmit pulses are commonly used which result in a narrower bandwidth fundamental. As discussed earlier, a longer transmit pulse (spatial pulse length) results in degraded axial resolution. For this reason, conventional harmonics generally have worse axial resolution and is generally not ideal for axial measurements (measurements made in depth) such as IMT measurements. Some systems now offer novel techniques to get around the narrow banding issue such as pulse inversion and pulse-modulated harmonics. In essence, these techniques allow for the separation of the fundamental and harmonic bandwidths without using a longer transmit pulse that results in degraded axial resolution.
The benefits of harmonic imaging are primarily a reduction of clutter artifacts. Since harmonic beams are inherently narrower than the fundamental beams, lateral resolution is improved. Additionally, since the beam intensity is generally low in the near field before the beam has converged to the focus, harmonic generation is generally low in the near field. Since most imaging artifacts result from strong reflectors in the near field (clutter signals), lower-level harmonic signals in the near field result in fewer imaging artifacts, improving image quality (Fig. 4.5). Again since harmonic generation drops precipitously with lower beam intensities (lower MI), using harmonics when trying to image very deep in a large patient is not recommended as the harmonic signal will offer inadequate sensitivity. In these deep cases, using fundamental imaging is the correct approach.


Fig. 4.5
(a) Fundamental. (b) Harmonic imaging . (c) Fundamental. (d) Harmonic imaging
Compound Imaging
Compound imag ing (known as multi-angle imaging , Sono CT or Crossbeam technology by some manufacturers) is a frame averaging technique, which can significantly improve image quality through increased signal-to-noise ratio and by decreasing the presence of artifacts. The angle dependency of ultrasound reflection is reduced by imaging the same region from different directions and “compounding” the resulting multiple frames into one image. Like all averaging techniques, the signal-to-noise ratio is improved when the desired signal does not change or changes slowly relatively to the rate of frame acquisition. In cases where the signal is changing slowly, the signal in each of the frames has the same phase such that adding together the frames results in the signal amplitude increasing by a factor equal to the number of samples. For example, adding four signals with an amplitude of 3 V, each would result in a combined signal with an amplitude of 12 V. In contrast, the noise which exists within the image is random from frame to frame, implying that although the noise amplitude also grows with averaging, it grows at a slower rate than the signal. In fact, the noise generally grows by the rate of the square root of the number of frames used in the average. Using the same example, imagine that the noise level is 1 mV (milli Volt), averaging four frames together would result in the noise becoming bigger by the square root of four, or 2 × 1 mV equal to 2 mV. Therefore, the signal grew four times larger, whereas the noise grew only twice as large, implying that the signal-to-noise ratio increased by a factor of 4 divided by 2, or 2 times. In other words averaging improves the signal-to-noise ratio by the square root of the number of samples in the average.
In addition to averaging, compound image varies the image steering from frame to frame. Recall that specular reflection is very angle dependent. Combining this fact with the fact that most imaging artifacts are caused by specular reflection, it should be clear that compound imaging results in artifacts tending to “average out.” The net result is that compound imaging generally results in images with better signal to noise (improved sensitivity) and fewer artifacts as seen in Fig. 4.6.


Fig. 4.6
(a) Conventional imaging . (b) Compound imaging
Also, because of the angle dependency of ultrasound reflection, by imaging from several angles, the circumference of arteries in cross section becomes more visible with compound imaging compared to single-angle imaging (conventional ultrasound imaging technique).
Image Optimization
Image optimization requires a comprehensive knowledge of the many system controls discussed above. There are no set rules which always govern when one technology should be applied or another disabled other than understanding the trade-offs as well as the underlying principles of each technology and control relative to the imaging situation. In general, the starting point for good image quality is to ensure adequate signal strength by appropriate transducer frequency selection, focal/foci depth, and imaging window. With respect to identification of artifacts, it is important to vary the incident angle (by electronically steering the image or physically angling the transducer) while looking for changes within the image. When the relative locations of structures vary with varying angle, imaging artifacts are present. Once the appropriate signal strength (signal-to-noise ratio) is achieved, the receiver gain and time gain compensation (TGCs) should be adjusted so that the full range of existing signals are visible relative to the ambient light and so that similar tissues at differing depths are displayed with similar brightness. Periodically, the compression should be adjusted to vary the contrast resolution so as to make certain that no signals such as fresh thrombus or masses are not being obscured by inappropriate contrast. Newer technologies such as harmonics and compound imaging often provide significant improvement but are at times inappropriate based on the trade-offs of the technologies. Finally, many systems now offer adaptive processing which attempts to perform image optimization based on internal algorithms. There are times when these adaptive processes will generate better image quality than even the most seasoned sonographer. However, there are other times when a talented sonographer will produce significantly better images than the adaptive processes. For this reason, the best use of these adaptive processes is to first optimize the image by hand and activate the adaptive process and then compare. It is then useful to optimize again by hand starting with the settings chosen by the adaptive processing technique. If the image only degrades by further manipulation, then reactivating the adaptive process will return the image to the “optimal” quality. By employing this approach, the sonographer can be pretty certain that he or she achieves optimal image qual ity.
IMT Measurements
In recent years carotid intima-media thickness (IMT) measurements have been used as an indicator for atherosclerotic disease with the intent of predicting likelihood of myocardial infarction and stroke. The ability to both accurately and repeatedly measure a vessel’s wall thickness is highly dependent on technique and ultrasound system settings. This section outlines those parameters which should be considered when performing IMT measurements . Realizing that equipment from different manufacturers will have different ideal settings, the purpose of this section is to make the reader aware of the potential issues, not to create a standard for measurement. Measurement standards require significant research and potentially may need to vary for different equipment design.
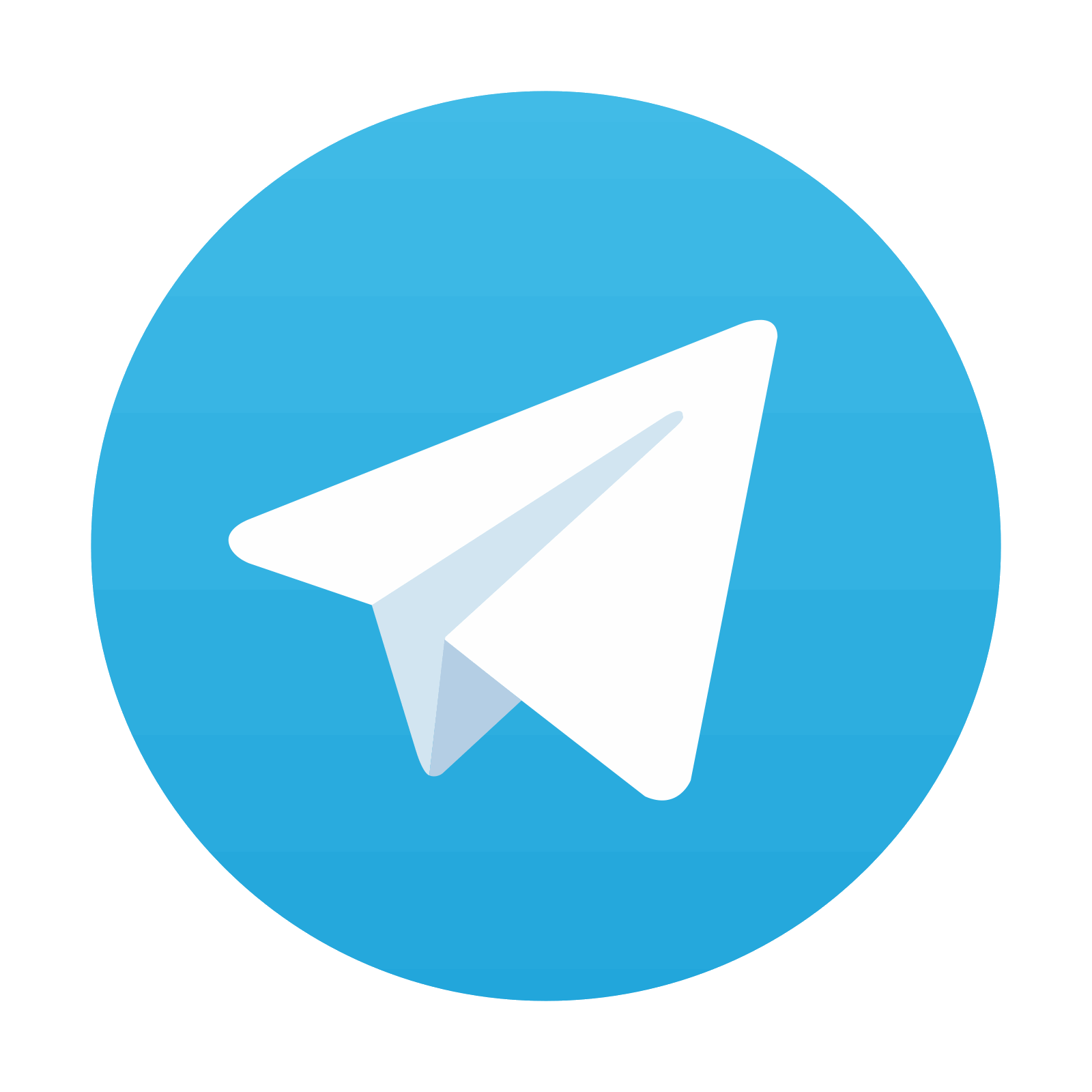
Stay updated, free articles. Join our Telegram channel
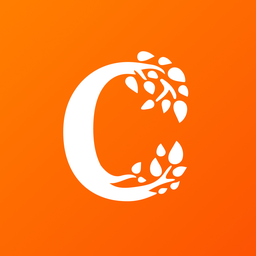
Full access? Get Clinical Tree
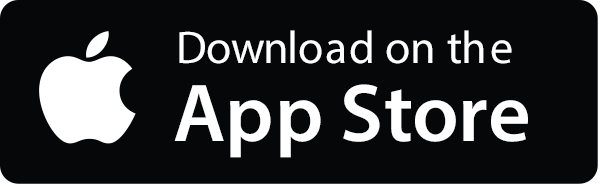
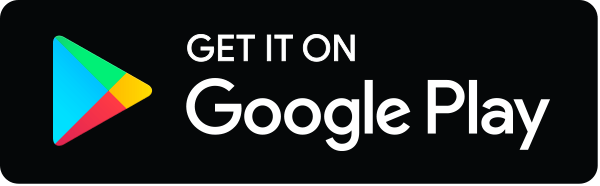