The TCS is a functional compartment represented by the amount of fluid and electrolytes continually exchanged (in and out) by cells with the ISS and by the IVS with the ISS. Other fluids composing the ECS are secretions, ocular fluid and cerebrospinal fluid (Agrò and Vennari 2013).


Fig. 40.2
Gamble gram. Electric neutrality principle: the sum of plasmatic cations is equivalent to the sum of plasmatic anions (Modified from Agrò et al. 2014)
The TBW represents about 90 % of total body weight in neonates. It decreases significantly during the first 6 months of life and reaches adult levels after 1 year of age. The ECS constitutes the main part of TBW and decreases in parallel from 40 % in term newborns to adult levels after 1 year of age (Suempelmann et al. 2013). The ECS expansion in neonates is mainly due to higher ISS fluid than adults. This becomes relevant as neonates have difficulty mobilizing fluid and electrolyte excess, potentially leading to pulmonary or peripheral oedema (Sulemanji and Vakili 2013). The composition of the ECS fluid and plasma is similar in neonates, children and adults, but dehydration occurs more rapidly in children because they need more fluids (Suempelmann et al. 2013) (Table 40.1).
Table 40.1
Composition of the ECS fluid, plasma and ICS fluid
Properties | Plasma | ISS fluid | ICS fluid |
---|---|---|---|
Colloid osmotic pressure (mmHg) | 25 | 4 | – |
Osmolality (mOsmol/kg) | 280 | 280 | 280 |
pH | 7.4 | 7.4 | 7.2 |
Na+ (mmol/l) | 142 | 143 | 10 |
K+ (mmol/l) | 4 | 4 | 155 |
Cl− (mmol/l) | 103 | 115 | 8 |
Ca2+ (mmol/l) | 2.5 | 1.3 | <0.001 |
Soon after birth, kidneys eliminate sodium and water excess in the ECS determining a redistribution of body water and the early postnatal weight loss (a decrease in 5–10 % of body weight). The site where most of the sodium exchange is done is in the distal tube (O’Brien and Walker 2014).
Expansion of the ECS by excessive administration of sodium and water, particularly before the postnatal diuresis has occurred, has an adverse effect on outcomes, especially in extremely low birth weight infants (Bell et al. 1980; Stephens et al. 2008). A Cochrane review of randomized controlled studies comparing liberal to restricted water (and sodium) intake in preterm neonates showed a significant increase in postnatal weight gain, in the risk of patent ductus arteriosus (PDA) and necrotizing enterocolitis, with an increased incidence of bronchopulmonary dysplasia, intracranial haemorrhage and death (Bell and Acarregui 2008). A retrospective chart review of 204 premature neonates (gestational age < 32 weeks) suggested that restricted water intake in the first 3 days of life (constant calorie intake) was protective for the development of PDA, with a statistical significance observed for analysis accorded for gestational age and severity of illness (Stephens et al. 2008). A randomized controlled trial in preterm newborns (gestational age <30 weeks) showed that early sodium supplementation (4 mmol/kg/day) was associated with delayed postnatal diuresis, delayed reduction in ECS water and increased oxygen requirement at 1 month (Hartnoll et al. 2000a, b). Moreover, excessive sodium administration may result in fluid retention, oedema and hypernatraemia, even in term neonates.
The ICS water does not vary much during infancy, from 30 % at birth to 40 % in adult (Friis-Hansen 1961). In adults, insensible water losses (IWL) consist mostly of water lost via evaporation through the skin (2/3) or respiratory tract (1/3). In neonates, IWL from the skin depend on gestational age: the more preterm the infant, the greater the transepidermal water loss. This is due to a higher body surface area/weight ratio and to a thin and fragile skin, poorly keratinized, especially in preterm neonates (Modi 2005). IWL may increase using a radiant warmer or phototherapy, determining a significant effect on fluid balance. In extreme preterm infants, IWL may exceed renal water losses. Evaporation of water from the skin is associated with cooling due to the effect of the latent heat of evaporation. Difficulty in keeping a baby warm may be a sign of excessive IWL. IWL may be reduced by nursing preterm infants <2 weeks of age in a heated humidified incubator (>80 % humidity). However, if the baby is taken out of the incubator (for instance, for surgery) or if the incubator is left open for procedures, this protection will be lost. IWL decrease as preterm neonates mature, and ambient humidity may be gradually decreased with time. In ventilated babies, humidification reduces IWL from the lungs. It is also required for babies receiving nasal CPAP or nasal ‘high flow’ therapy. Postextubation, respiratory IWL may be high if a neonate receives unhumidified O2 via nasal cannulas. IWL cannot be measured but should be estimated to allow for appropriate fluid prescription. IWL can be estimated using the following formula (Jansen et al. 2012):


Fluid and Solute Movements
Fluid and electrolyte balance is both an external balance between the body and its environment and an internal balance between the ECS and ICS, and between the IVS and ISS. This balance is based on specific chemical and physical properties of body fluids, such as ionic composition, pH, protein content, osmotic pressure, osmolarity and colloid osmotic pressure, leading water and solutes to move across the compartments (Agrò and Vennari 2013). Body compartments are surrounded by a semipermeable membrane through which fluids and solutes selectively pass according to the properties of the fluids (Musso et al. 2004). Moreover, water and solute movement between the IVS and ISS is regulated by the capillary endothelium and the overlying capillary endothelial glycocalyx, which together form the endothelial glycocalyx layer (EGL). The glycocalyx consists of glycoproteins and proteoglycans containing glycosaminoglycans attached to the endoluminal surface of the capillary endothelium. It is a dynamic structure continuously degraded and resynthetized, impermeable to large molecules (>70 kDa), and probably it is the main responsible for the oncotic gradient across IVS and ISS. Albumin is contained within the EGL, and normal plasma albumin levels are required to assure EGL functions (Young 2012). Furthermore, glycocalyx prevents the endothelial adhesion of inflammatory cells, reducing the risks of an increased endothelial permeability. A glycocalyx damage leads larger molecules to pass from the IVS into the ISS, reducing the IVS–ISS oncotic gradient and increasing the ISS volume with tissue oedema. Various conditions associated to cardiac surgery may potentially destroy glycocalyx: hemodilution, ischaemia and reperfusion damage and inflammation (Young 2012).
Electrolyte Balance
Ionic balance is based on the principle of the ‘electric neutrality’: the sum of cations must be the same of the sum of anions. In other words, the net sum of the electric charge in the body fluids is zero. Ionic composition of ICS and ECS is different, and further differences exist in the ECS between the IVS and the ISS (Table 40.1). In clinical practice, the only value directly measurable is the plasmatic concentration of each ion. Generally, this value is considered as a reference to evaluate the presence of electrolyte alterations. The relationship between the ionic plasmatic composition and the neutrality principle is expressed by Gamble gram (Fig. 40.2). Examining the Gamble gram is immediately evident that the sum of cations (Na+ + K+ + Ca++ + Mg++ + others) is 154 mEq/L and is the same of anions (Cl− + bicarbonate + proteins + phosphates + sulphates + organic acids). Na+ and K+ represent the 94 % of all IVS cations, while Cl− and bicarbonate represent the 84 % of all anions. Na+, K+, Ca++ and Mg++ are electrolytes generally measured through laboratory exams, while bicarbonate is only calculated using Henderson–Hasselbalch equation when arterial blood sample is performed (Gamble 1947).
Sodium
Sodium is the most highly represented cation in the ECS and it has a key hemodynamic role: it is the main determinant of ECS volume, contributes to renin–angiotensin–aldosterone system (RAAS) activation and regulates ADH secretion. Sodium concentration determines body fluid osmolarity. Changes in sodium plasma level are responsible for modification in fluid movement across the body space, determining ICS and ECS volume variation. The normal sodium concentration in plasma and the ISS is about 142 mEq/L, and it is higher than the ICS concentration (10 mEq/L) (Agrò and Vennari 2013). Neonates are susceptible to sodium disorders, and both sodium content and water amount administered through IV fluids should be considered carefully. Aldosterone secretion is slow to be reduced in the face of a sodium load, for instance, from isotonic fluid boluses, intravenous flushes and drugs, and may result in hypernatraemia or sodium retention with oedema formation. It is recommended that neonates are given sodium-free fluids until after the postnatal diuresis to allow for contraction of the ECS volume (O’Brien and Walker 2014), but inadequate sodium intake thereafter will result in hyponatraemia (Hartnoll et al. 2000a, b). This is particularly important in preterm neonates as the renin–angiotensin–aldosterone system (RAAS) is less active, causing a limited ability to retain sodium in the distal renal tubule. Inadequate sodium intake is associated with severe hyponatraemia and poor long-term neurological outcomes in preterm neonates (Baraton et al. 2009).
Potassium
Potassium is the main cation of the ICS. It plays a central role in determining the resting cell membrane potential, especially for excitable cells such as myocytes. Therefore, it influences the transmission of impulses along the cardiac pacemakers (potentially predisposing to arrhythmias) and the contraction of myocardial cells. It is also involved in a variety of metabolic processes, including energy production and the synthesis of nucleic acids and proteins (Agrò and Vennari 2013). The kidney plays an important role in maintaining potassium balance in the body. Potassium is freely filtered by the glomerulus and reabsorption occurs in the proximal tubules. There is some reabsorption in the ascending loop, but the final urinary concentration is determined by the secretion in the distal tubule. In premature neonates, hyperkalaemia is usually evident due the immaturity of the distal tubules. The peritubular and luminal permeability to potassium may also contribute to the physiologic positive balance. A major determinant of potassium balance is cellular metabolism. There is a shift of potassium from the ISS to the ECS immediately after the birth in preterm infants (Lorenz et al. 1997). Once the kidney adapts to the extrauterine environment, the increased diuresis facilitates potassium excretion and the regulation of serum potassium levels.
Calcium
Calcium balance in the body is maintained by a well-coordinated mechanism between the gastrointestinal tract, bone and kidneys. The kidneys regulate calcium reabsorption throughout the nephron via various active and passive processes. Calcium is involved in endocrine, exocrine and neurocrine secretion, coagulation activation, muscle contraction (it has a great inotropic effect), potential membrane depolarization, cell growth and enzymatic regulation and in the metabolism of other electrolytes (especially potassium and magnesium). Calcium may circulate in the plasma bound to albumin and free from proteins. Free calcium may be ionized (physiologically active) or nonionized (chelated with inorganic anions such as sulphate, citrate and phosphate). The amounts of the three forms are altered by many factors, such as pH, plasma protein levels (hypoalbuminaemia reduces total calcium, but not free fraction) and percentage of anions associated with ionized calcium (blood products contain citrate) (Agrò and Vennari 2013). Although there is a strong correlation between serum total calcium levels and serum-ionized calcium, total calcium can be a poor predictor of calcium status especially in neonates. Low levels of calcium are common in premature infants, but seldom results in tetany or decreased cardiac contractility (Venkataraman et al. 1985). Calcium levels tend to stabilize and reach childhood levels by the first week of life (Sulemanji and Vakili 2013).
Phosphorus
Phosphorus is similarly and concordantly regulated with calcium. It has an important role in bone structure and various metabolic processes. The normal plasma phosphorus level is maintained through a balance between intestinal absorption versus renal excretion. Renal excretion is the primary mechanism by which phosphorus is regulated in the body. Parathyroid hormone (PTH) is the most potent hormone that controls urinary excretion of phosphorus. Elevation in phosphorus levels induce the secretion of PTH, which in turn leads to the secretion of phosphorus via the kidneys. Excess phosphorus develops a complex with calcium resulting in a decrease in the production of calcitriol, thereby reducing calcium absorption in the gut (Agrò and Vennari 2013).
Magnesium
Magnesium is the physiological antagonist of calcium. It plays a crucial role in neuromuscular stimulation and modulation of excitable cell activity (membrane-stabilizing activity); it also acts as a cofactor of several enzymes involved in the metabolism of three major categories of nutrients: carbohydrates, lipids and proteins (Agrò and Vennari 2013).
Chloride
Chloride is the most important anion of the ECS. Together with sodium, it determines the ECS volume, and it plays a crucial role in acid–base balance (SID approach). It is also responsible for the resting potential of the membrane and action potential, and plasma osmotic pressure (Agrò and Vennari 2013).
Bicarbonate
Bicarbonate is the main buffer system of the blood. It plays a critical role in maintaining acid–base balance. Two-thirds of the CO2 in the human body is metabolized as bicarbonate, through the action of carbonic anhydrase. The equilibrium between CO2 and bicarbonate leads to the elimination of volatile acid. The bicarbonate buffer system is described by the following equilibrium reaction:
When there is an increased concentration of H+, the system reacts by shifting the reaction equilibrium to the left (towards the production of CO2); while when the concentration of H+ is reduced, the system moves to the right, resulting in the production of H+. The bicarbonate buffer system works ‘in concert’ with several organs. Bicarbonate has a normal plasma concentration of about 24 mmol/L (Agrò and Vennari 2013).

Acid–Base Balance
The acid–base balance is regulated by a combination of the respiratory, buffer and renal systems. The buffer system constitutes the bicarbonate–carbonic acid buffer, haemoglobin–oxyhaemoglobin buffer, protein buffer and the phosphorus buffer mechanisms. The buffer systems are adapted to serve as the primary mechanism for maintaining acid–base balance in the newborns (Sulemanji and Vakili 2013). With low serum bicarbonate levels and ongoing physiologic demands, the premature infants have a tendency to acidosis. It is known that administration of bicarbonate gives little benefit in comparison of risks, including intraventricular haemorrhage, deteriorating cardiac function and the worsening of intracellular acidosis (Aschner and Poland 2008). However, if strictly needed, bicarbonate should be administered at a very slow rate in order to minimize fluctuations in cerebral hemodynamics (Berg et al. 2010). Term neonates, with the exception of those having congenital complications, usually have a stable physiologic transition from foetal life to extrauterine surrounding. Cardiovascular, respiratory and cerebral hemodynamic mechanisms are in equilibrium with each other and generally result in a balanced acid–base homeostasis. The acid–base balance is maintained closely by complex interactions between the respiratory system and the kidneys. The acceptable values in term infants compared to preterm infants (o28 weeks) are as follows: pH > 7.30 (>7.28), PaCO2 40–50 (40–50), bicarbonate (HCO3) 20–24 (18–24) and PaO2 50–70 (50–65). The respiratory effort in the term infants is almost always stable with marginal predisposition to respiratory acidosis. At the same time, the buffer systems and tubular handling of the term infant kidney are also mature to handle any non-respiratory-induced acidosis within 72 h following birth (Malan et al. 1965). Classically, there have been three different clinical approaches to acid–base physiology and management:
The descriptive approach
The semi-quantitative approach
The quantitative approach
The first is mainly founded on Henderson–Hasselbalch equation, the second on base excess (BE) and the third on strong ion difference (SID) (Fig. 40.3). They use distinct variables derived from a set of master equations that can be transferred from one approach to the other two (Agrò and Vennari 2013; Kellum 2005):


Fig. 40.3
The three possible approaches to acid–base balance system description. Some factors (i.e. pCO2) are considered by all the approaches (Modified from Agrò and Vennari 2013, pp 1–26)
Renal Physiology
Renal function is related to the maturation and size of the nephrons, which have the ability to filter the blood and to collect the filtrate. At birth, the kidneys are still undeveloped with reduced ability in reabsorption. Thus, newborns cannot concentrate urine as effectively as adults, and they are unable to excrete large salt loads. After 1 month, the kidneys reach about 60 % of their maturation, but the reabsorptive capacity remains lower than in adults. In the first 2 years, the maturity and function of the kidneys increase greatly and reach adult levels (Suempelmann et al. 2013; Bissonnette Bruno 2011).
Renal Blood Flow
Renal blood flow (RBF) changes throughout the years from newborns to adults. It is influenced by the ratio of renal/systemic vascular resistance and the cardiac output (CO). RBF is only about 3–7 % of the CO in the foetus (Rudolph and Heymann 1968). After the birth, it is improved consequentially to a reduction in renal vascular resistances and an increase in CO. In the first week of life, RBF is only the 10 % of CO. In the neonate, the relation between RBF and kidney weight, body weight and surface area is lower than the adult (Sulemanji and Vakili 2013). From childhood to adults, a combination of increased renal perfusion pressure and decreased renal vascular resistance leads to an improvement of RBF up to 25 % of CO (Musso et al. 2004). The clearance of p-aminohippurate has traditionally been used to measure the effective renal plasma flow (ERPF). The ERPF has been reported as:
<20 mL/min/1.73 m2 in the premature infant
45 mL/min/1.73 m2 by 35 weeks of gestation
83 mL/min/1.73 m2 in term infants (Musso et al. 2004)
It progressively increases to reach 300 mL/min/1.73 m2 by toddler age and finally reaches adult rate of 650 mL/min/1.73 m2 by 2 years of age. This increase is associated with a proportionally higher flow to the outer cortical region. At any age, ERPF may be modified by renal flow autoregulation. However, autoregulation is less efficient in infants, especially at lower baseline values as in newborns (Musso et al. 2004).
Glomerular Filtration Rate
The determinants of glomerular filtration rate (GFR) are:
Starling forces across the capillary wall
Permeability of the glomerular wall
Total surface area of the capillaries
RBF (Fig. 40.4)
Fig. 40.4
Determinant of glomerular filtration. Pf filtration pressure, Pc hydrostatic pressure in the capillary (glomerulus), πp colloid osmotic pressure in Bowman’s capsule, PB hydrostatic pressure in Bowman’s capsule
GFR is established during intrauterine life, but it is insignificant because the kidneys do not primarily function as a water- and fluid-regulating organ. After birth it significantly increases, as the kidney assumes its role in fluid, water and electrolyte balances (Yared 2004). In the newborn, GFR is about 40 mL/min/1.73 m2 and it reaches 66 mL/min/1.73 m2 by 2 weeks of age. Adult levels of 100–125 mL/min/1.73 m2 are reached at around 2 years of age (Schwartz et al. 1987). In addition, maximal urine concentration capacity of the term infants (700 mOsm) does not reach adult levels (1400 mOsm) until 6–12 months of age (Rudolph and Heymann 1968). In very low birth weight (VLBW) infants, the increase in GFR is generally reduced than normal weight infants, reaching normal levels later in childhood. Neonates, especially premature, have a limited ability to handle fluid loads: variations in GFR are clinically relevant because they affect fluid and electrolyte homeostasis as well as excretion of drugs (Iacobelli et al. 2007).
A variety of mechanisms are involved in GFR regulation. First of all, macula densa cells of the tubuloglomerular system regulate GFR according to the rate of distal tubular flow and the chloride concentration of the tubular fluid: a high chloride concentration is interpreted as an elevated GFR, while a low chloride concentration as a low GFR. Macula densa cells regulate the afferent arteriole and glomerular capillary tone leading to an adjustment in the GFR (Koeppen and Stanton 2004) (Fig. 40.5). The myogenic reflex regulates GFR based on renal perfusion pressure. Decreased perfusion pressure leads to a dilation of the afferent arteriole and vasoconstriction of the efferent arteriole (Koeppen and Stanton 2004) (Fig. 40.5).


Fig. 40.5
The tubuloglomerular system and the myogenic reflex
Renal perfusion pressure is firstly compromised in many clinical settings such as cardiac dysfunction, hypovolaemia or septic shock. In any case (normal, reduced or increased intravascular volume), GFR is maintained by an increase in the filtration fraction in the context of diminished CO and RBF. The myogenic reflex is initially adequate, but as cardiac function continues to deteriorate, it no longer can maintain an acceptable GFR. As a consequence, GFR decreases secondary to diminished renal perfusion leading to accumulation of water and solutes via multiple sodium-retaining systems. Water- and salt-retaining mechanisms are used by kidneys to restore CO and arterial pressure. Depending on the nature and degree of CO reduction, the retention may lead to a vicious cycle of worsening oedema and congestion. However, a drastic RBF decrease may overwhelm the kidney’s ability to autoregulate, resulting in a dramatic GFR diminution. When a mild to moderate reduction of renal perfusion develops, GFR may be maintained via various mechanisms that act on the afferent (vasodilator prostaglandins) and efferent (angiotensin II) arteriolar systems. However, any aggravation of this system by exogenous factors (ACE inhibitors and/or NSAIDs) may produce an important fall in the GFR (Ricci et al. 2011a).
The renin–angiotensin–aldosterone system (RAAS) is activated by secretion of norepinephrine in the peripheral vessels. The RAAS action determines intrarenal vasoconstriction and subsequently diminished RBF with increased sodium retention (DiBona and Sawin 1991, 1995; DiBona and Kopp 1997) (Fig. 40.6). The RAAS is responsible for regulating blood pressure, RBF, fluid and electrolyte balance. Renin is the key component of the system. It is produced in the kidney by the juxtaglomerular cells. The mechanism controlling release of renin is well established by late gestation. Hypotension, haemorrhage, furosemide, ACE inhibitors, prostaglandins, vasopressin and atrial natriuretic peptide are all known factors that influence renin secretion. Renin triggers the formation of angiotensin I (ATI) which subsequently gets converted to angiotensin II (ATII) by angiotensin-converting enzyme (ACE). ATII, through plasma membrane receptors ATI and ATII, increases systemic blood pressure determining a vasoconstriction of small vessels with an increase in peripheral resistances. Furthermore, ATII can increase CO through increasing myocardial contractility (Sulemanji and Vakili 2013). ATII has an autocrine effect: it is the primary vasoconstrictor of the renal vessels, modulating reabsorption of sodium and water by kidneys (Kobori et al. 2007). ATII renal action is greatest on the efferent arteriole than afferent, leading to an increase in the filtration fraction with retention of water and sodium (Ichikawa et al. 1984). Moreover, the release of ATII leads to the production and secretion of aldosterone by the zona glomerulosa of the adrenal gland. Aldosterone acts on the mineral corticoid receptors of the kidney, heart, brain, colon and vessel walls. Aldosterone effect on kidney determines sodium retention, which eventually leads to retention of water (Garty 1992).


Fig. 40.6
Renin–angiotensin–aldosterone system: hypovolaemia reduces perfusion of juxtaglomerular apparatus, with renin release. Circulating renin converts angiotensinogen to angiotensin I, subsequently angiotensin-converting enzyme (ACE) acts on angiotensin I converting it to angiotensin II. This hormone increases NSS activity, increases reabsorption of Na and water by kidneys directly and through aldosterone action and determines vasoconstriction and ADH secretion (Modified from Agrò and Vennari 2013, pp 71–92)
Components of RAAS are present during early gestation, but their activity and function are somewhat different than adults. RAAS is active in the kidney prior of foetal urine production. This may suggest a role in regulating growth and development of the nephron. At birth, plasma renin activity is increased and continues to stay elevated through infancy. It begins to decline to adult levels by 6–9 years of age (Stalker et al. 1967). Both ATI and ATII receptor expression increase exponentially after birth (Tufro-McReddie et al. 1993), contributing to vasoconstriction of the neonatal kidney. Likewise, there is augmented production of renin, AT and ACE in the postnatal kidney. These effects are counteracted by the postnatal increase in prostaglandins, nitric oxide and kinins which promote vasodilatation and contribute to the maturational increase in RBF (Carey et al. 2000). When CO is reduced, prolonged sodium retention persists with subsequent accumulation of extracellular water. Moreover, a clinical study showed a severe reduction of GFR in young rats VS a mild reduction in adults, when renal perfusion pressure is decreased by 30 % (Yared and Yoshioka 1988). Micropuncture experiments revealed that the young rats had decreased glomerular capillary pressure due to incompetence of the ATII-mediated vasoconstriction effects: the young rats may not be able to activate ATII, and the immature efferent arteriole may not respond to ATII the same way as in adults. This is not due to receptor and angiotensin availability but instead to immature post-receptor processes (Yared and Yoshioka 1988).
Aldosterone is one component through which angiotensin regulates sodium reabsorption, influencing fluid and electrolyte balance. The foetal response to secrete aldosterone is less than that seen in adults due to the relative insensitivity of the adrenal gland (Robillard et al. 1982). It is well known that infants with poor cardiac function secondary to congenital heart disease are at risk for acute kidney injury. Mechanoreceptors in the aortic arch, left ventricle and renal afferent arterioles sense systemic arterial pressure and regulate IVS volume. Arterial underfilling activates the sympathetic nervous system with an increase in myocardial contractility, heart rate and peripheral and renal vasoconstriction. Stimulation of the RAAS also contributes to systemic vasoconstriction as well as vasoconstriction of the efferent and afferent arterioles mediated by AT II. Sympathetic stimulation and ATII increase sodium transport in the proximal tubule and deliver less sodium to the distal tubule. This leads to persistent aldosterone-mediated sodium retention in the collecting duct (Fig. 40.7). Moreover RAAS activation can have deleterious effects on the heart. In fact, aldosterone increases myocardial collagen deposition, fibrosis, inflammation and remodelling of the heart and blood vessels (Schrier et al. 2010). ATII additionally contributes to left ventricular hypertrophy as well as remodelling (Booz and Baker 1996).


Fig. 40.7
Mechanism of ADH secretion: when fluid volume decreases, plasma sodium concentration and plasmatic osmolarity increases, leading to hypothalamic osmoreceptor stimulation. The hypothalamus will then stimulate the posterior pituitary gland that releases antidiuretic hormone. ADH will make renal distal tubules able to reabsorb water into the IVS in order to maintain homeostasis of fluid balance. ADH secretion is more sensible to plasmatic osmolarity than circulating blood (Modified from Agrò and Vennari 2013, pp 71–92)
The antidiuretic hormone (ADH) is secreted from the posterior pituitary in response to severe arterial underfilling leading to osmolarity modification. Stimulation of ADH receptors leads to the expression of aquaporin-2 water channels on the apical surface of the collecting duct, resulting in an increase of water reabsorption restoring osmolarity and volume of IVS (Fig. 40.7). The persistent activation of these adaptive mechanisms leads to fluid overload, worsening heart failure and decreased renal perfusion (Funayama et al. 2004; Pedersen et al. 2003).
The atrial natriuretic peptide (ANP) increases GFR by constriction of efferent arteriole and dilatation of the afferent arteriole (Marin-Grez et al. 1986). ANP also acts on sympathetic renal effect: it may reverse sympathetic-induced afferent vasoconstriction and potentiate efferent arteriolar vasoconstriction. These effects may suggest a role of ANP in maintaining GFR in heart failure patients, in which ANP values are elevated and renal perfusion pressure is reduced. In addition, ANP counteracts the effects of ATII on the proximal tubule in regard to sodium and water retention: it promotes natriuresis by inhibiting tubular sodium reabsorption (Harris et al. 1987). Moreover, ANP inhibits renin secretion and reduces aldosterone secretion by the zona glomerulosa of the adrenal cortex. It also counteracts maladaptive cardiac hypertrophy and remodelling mechanisms.
The brain natriuretic peptide (BNP) is produced mostly in the ventricular myocardium and has similar action respect with ANP. ANP and BNP react to the effects of the RAAS and sympathetic activation seen in acute cardiac dysfunction. Studies have shown that BNP secretion increase after a left ventricular dysfunction (Wei et al. 1993). BNP’s effect is similar to those of ANP.
Prostaglandins are potent renal vasodilators produced by arachidonic acid in many cells throughout the body. The major action of prostaglandins is to modulate the actions of vasoconstrictors.
Nitric oxide is an endothelium-derived gas synthesized from the amino acid L-arginine by nitric oxide synthase. It diffuses across the endothelial membrane and enters vascular smooth muscle cells, inducing vasodilatation. In the kidneys, the afferent arteriole is more sensitive than the efferent arteriole to the vasodilator effects of NO. The main action of NO is to modulate the action of angiotensin.
Urine Concentration and Sodium Excretion Fraction
The ability to maintain a negative water and sodium balance through a high sodium excretion is inversely proportional to the infant’s maturity. As a result, this process results in a greater loss and lasts longer in premature infants.
After the postnatal diuresis, the neonate grows rapidly. Renal function is ideally adapted to cope with a liquid (milk) diet with relatively low sodium content. Sodium is required for growth and is retained avidly in the distal tubules under the influence of the renin–angiotensin–aldosterone system (RAAS). Although the kidney has a full complement of nephrons from around 35 weeks’ gestation, the renal tubules are short, and there is limited ability to concentrate the urine (Haycock 2005). High volumes of dilute urine are therefore produced (urinary osmolarity 300 mOsmol*kg−1) at a rate of around 2–3 mL*kg−1*h−1 (45–50 mL*kg−1*day−1). Neonates are also able to produce more dilute urine in the face of a high water load (provided ADH levels are not elevated), but they have limited ability to concentrate the urine and so become dehydrated easily (Bell and Acarregui 2008). Growth of the kidney is associated with increasing complexity and length of the renal tubules and increasing ability to concentrate the urine, so that by a year of age, infants are able to vary the concentration of urine between 50 and 1400 mOsmol/kg, as in adults (Haycock 2005).
Fractional excretion of sodium (FE Na) is highest during the first 10 days of life and decreases to below 0.4 % by 1 month of age which is comparable to adults. FE Na may increase secondary to hypoxia, respiratory distress, hyperbilirubinaemia, increased fluid or salt intake and diuretic administration (Jose et al. 1994). The major regulators of FE Na are the renin–angiotensin–aldosterone system, atrial natriuretic peptides, prostaglandins and catecholamines (Nafday 2005). It is the balance between these factors that compensates for the immaturity of the nephron in order to maintain systemic homeostasis.
Acute Kidney Injury After Congenital Heart Disease Surgery
Congenital heart disease surgery is one of the most common causes of renal failure. In fact, adult and paediatric cardiac surgery is complicated in 30–40 % of cases by acute kidney injury (AKI), and it is associated with adverse outcomes, including diminished quality of life, prolonged intensive care and hospital stays and increased long-term mortality, particularly in patients who require dialysis. Furthermore, even minor degrees of postoperative AKI caused a significant increase in mortality and morbidity.
Epidemiology of AKI
The incidence of AKI after cardiac surgery in both paediatric and adult patients may be widely variable because of the shortage of standardized definitions. It has been shown that AKI has an incidence of 7.2–48 % with a mortality of 14–80 % (Kuitunen et al. 2006) in adult postoperative cardiac patients, depending on the definition used and the population analysed. Moreover, AKI is the strongest independent risk factor for mortality (Lassnigg et al. 2004), following adult cardiac surgery. In paediatric patients, the incidence of AKI after congenital heart surgery varies in a wide range between 3 and 61 % with mortality from 20 to 79 % (Picca et al. 1995; Aydin et al. 2012). Price et al. (Price et al. 2008) linked a worsening in renal function (increase in Scr > 0.3 mg/dL) with acute decompensate heart failure. They found an incidence of worsening renal function in 48 % of patient with acute decompensated heart failure, and it was related with in-hospital death or requirement for mechanical circulatory support.
Aetiology and Pathogenesis of AKI Post-Cardiac Surgery
A lot of factors are essential in the development of AKI after cardiac surgery. In fact, the pathogenesis is related to the complex interaction between tubular, vascular and inflammatory factors. It is well known as, both in child and adult patients, AKI after cardiac surgery mainly caused by acute tubular necrosis (ATN). Microscopically, ischaemia (e.g. as a result of CPB, low cardiac output, circulatory arrest) produces an ATP depletion that activates oxidative and cell death mechanism, with a loss of brush borders and disruption of cell polarity and cytoskeleton. These lead to cell apoptosis and, gradually, to a desquamation of tubule cells into the lumen, causing a cast obstruction. Moreover, the ischemic insult modifies the renal blood flow, causing hypoperfusion of the outer medulla. In this manner, the microvasculature becomes both a ‘source of’ and a ‘target for’ the inflammatory injury present in the ischemic kidney (Devarajan 2006). Injured endothelia and tubule cells are part of an inflammatory response which involve the systemic inflammatory response syndrome (SIRS) existing during and after CPB. Furthermore, these events may be exacerbating during reperfusion (see later). It is important to consider that, according to a low mean arterial pressure and high renovascular resistance, normal infants have a lower GFR, making the infant kidney weaker in than adult patients. Several factors may contribute to the failure of this mechanism in congenital heart disease paediatric patients than adults. Hypoxaemia, resulting from preoperative cyanosis or postoperative pulmonary impairment, reduces RBF and GFR, inducing hypotension, hypervolaemia and activation of the RAAS.
Frequently, patients need intubation and mechanical ventilation both in pre- and postoperative phase. Positive pressure ventilation impairs renal function because of it decreases the venous return and consequently the cardiac output, with an increase in renal sympathetic nervous activity and serum vasopressin levels.
Hypothermia, induced in operation theatre, causes renal vasoconstriction and a decrease in GFR.
As previously described, the angiotensin II activity plays a central role in the maintenance of GFR, because of causing vasoconstriction of post-glomerular, efferent arteriole. Prostaglandin synthase inhibitors, such as indomethacin, used in patient with patent ductus arteriosus, may blunt the vasodilatation of afferent arterioles needed to maintain adequate perfusion of the newborn kidney. Moreover, compared with adults, paediatric patients are more sensitive to the use of inhibitors of ACE inhibitors. The use of nephrotoxic drug or contrast in pre- and postoperative phase may induce directly tubular injury (Picca et al. 2008).
Postoperatively, sepsis may contribute to renal damage, because it induces an inflammatory response that leads to a production of vasoactive mediators that may worsen GFR and precipitate a heart failure.
All these mechanisms contribute to cell death, resulting in necrosis and/or apoptosis and even in patient death. A recent case–control study has been conducted to identify independent risk factors for AKI and dialysis. Among them are preoperative use of mechanical ventilation, milrinone, or gentamicin, intraoperative milrinone or furosemide, duration of anaesthesia, multiple cross clamps and transfusion of blood products (Chiravuri et al. 2011). As mentioned before, a lot of studies show that preoperative state of the congenital heart disease patient may also influence the development and the prognosis of AKI. Preoperative conditions favouring AKI are central venous hypertension, systolic arterial hypotension, pump failure or low cardiac output syndrome, use of inotropic and vasopressor drugs, high-risk complex operation, cyanotic cardiac disease and circulatory arrest (Pedersen 2012). Several of the risk factors are mutually related to each other, and identification of independent risk factors is dependent on the specific variables (Fig. 40.8). One of most important factors that may affect renal function, however, remains CPB.


Fig. 40.8
Main risk factors of post-cardiac surgery AKI
CPB and Ultrafiltration
Historically, CPB was associated with a multiorgan dysfunction that may result in increase in morbidity and mortality in patients who underwent cardiac surgery, particularly in the smallest neonates after repair of complex congenital heart defects. This is due to hemodilution and hypothermia, often in association with tissue ischaemia. Moreover, CPB may increase TBW, which participate to organ dysfunction and tissue oedema. Nowadays, improvements in the technology of CPB and use of ultrafiltration have significantly reduced morbidity after cardiac surgery.
Physiopathology
It is well known that patients undergoing cardiac surgery without CPB have preserved renal function and a milder systemic inflammatory response as compared to those requiring CPB (Ascione et al. 1999). This evidence is particularly strong in younger patients (especially neonates), as a result of their small body surface area. Two are the underlying causes of renal impairment: the hemodynamic changes during CPB and the activation of immune response. First, there is an association between CPB duration and the risk of AKI in infants (Picca et al. 1995). The non-pulsatile flow modifies the arterial resistance, with subsequent alteration in organ perfusion. This suboptimal perfusion may not compensate kidney oxygen consumption, resulting in ischaemia and initial organ damage. Reperfusion may worsen ischaemia, inducing cell death and inflammation, resulting in tissue damage and eventually organ dysfunction. Moreover, ischaemia and alteration in blood flow activate the RAAS, leading to fluid accumulation and initial fluid retention. The mechanic and ischemic insult produced by CPB cause also a systemic inflammatory response. First of all, blood components are exposed to the foreign surface of the CPB circuit. Per se, this can cause an inflammatory response (Abu-Omar and Ratnatunga 2006; Asimakopoulos 2001). In fact, an activation of the complement system and a secretion of various proinflammatory cytokines such as IL-8, IL-10 and TNF-ά have been found in patients who underwent CPB (Abu-Omar and Ratnatunga 2006; Asimakopoulos 2001). Furthermore, the production of proinflammatory mediators is stimulated by renal ischaemia. Particularly, the activation of the complement system plays a central role: it may cause a direct injury to the renal tubular epithelial cells and may promote secretion of cytokines, which worsen the epithelial damage. The oxygen-free radicals released from the ischemic tissue may contribute to end-organ damage. Finally, CPB increases the activation of neutrophils, monocytes and endothelial cells which have also been implicated in the development of kidney injury.
Ultrafiltration
One of the most important findings after CPB is a fluid overload (FO), which typically is more prominent in neonates (Berdat et al. 2004). Haemodilution, as a result of the large circuit priming volumes, may be associated with vital organ dysfunction and tissue oedema (Apostolakis et al. 2010). Moreover, a large volume and blood component shifts have been demonstrated in patients with congenital heart disease undergoing surgery with CPB, due to the inflammatory response and the production of vasoactive mediators that increase capillary permeability (Rosner and Okusa 2006). Miniaturization of CPB circuit (Merkle et al. 2004) and the use of intraoperative ultrafiltration are improvements that could have a significant impact on the prevention of AKI during CPB. Ultrafiltration (also known as haemoconcentration) is a variety of membrane filtrations in which water and low molecular weight solutes are removed from plasma, throughout a semipermeable membrane, by a transmembrane pressure gradient (a process known as solvent drag), so that the composition of the ultrafiltrate is related to the pore size of the haemofilter. The first application of ultrafiltration for the CPB was during rewarming, the so-called conventional ultrafiltration (CUF). With this technique, the ultrafilter (UF) of the perfusate is performed, while the patient is still being assisted by the lung–heart machine and the haemoconcentrator is connected to the recirculation line between the oxygenator and the venous reservoir. The volume of the venous reservoir of the bypass circuit restricts the volume of filtrate that can be removed during CUF, providing only a limited ability to remove excess water and reverse hemodilution (Cooper et al. 2011). For this reasons, Naik and colleagues (Naik et al. 1991a, b) introduced an ultrafiltration technique after separation from CPB, which they termed modified ultrafiltration (MUF). The difference is that blood is removed from the arterial cannulas and passed through a haemoconcentrator. Blood remaining in the venous reservoir is ultrafiltrated, haemoconcentrated and then returned to the right atrium (so-called arteriovenous MUF). A roller pump maintains a flow rate of approximately 200 mL/min with a filtration rate of 150 mL/min. Typically, the duration of ultrafiltration is approximately 20–30 min, but has a high institutional variability. Haemoconcentration is carried out until a haematocrit value of 40 % is achieved or no blood remains in the bypass circuit. A similar process, called veno-venous MUF, has been used too. Blood is withdrawn from the right atrium and returned to the right atrium. No direct comparisons of the effectiveness of arteriovenous MUF and veno-venous MUF have been performed. Various advantages are associated with the use of MUF. In a lot of studies, it has been demonstrated that MOF decreases FO, postoperative blood loss and blood product use (Naik et al. 1991b); improve left ventricular systolic function, with an increase in arterial blood pressure (Naik and Elliott 1993); improve alveolar–arterial oxygen gradient and lung compliance and decrease the frequency of pulmonary hypertensive episodes (Davies et al. 1998); decrease the duration of postoperative ventilation (Koutlas et al. 1997); and decrease the incidence of pleural effusions after cavopulmonary connection and the Fontan procedure (Koutlas et al. 1997). It has not been well elucidated the mechanism by which MUF causes these beneficial effects. Initially, the benefit appeared to be caused simply by removing FO decreasing tissue oedema. Subsequent studies showed a large amount in the ultrafiltrate of inflammatory mediators and vasoactive substances, including IL-6, IL-8, and IL-10, TNF-ά and endothelin 1 (Elliott 1999), so that early removal of the mediators may decrease the inflammatory cascade (Bando et al. 1998). As mentioned, CUF and MUF are two different processes, not competing, but can be complementary techniques with potentially additive positive effects. Filtration during CPB (CUF) may be used to remove inflammatory mediators and vasoactive substances, whereas MUF is performed after CPB to reverse haemodilution and decrease tissue oedema. Nowadays, the improvement in technology (small circuits, decrease use of haemodilution, less use of hypothermia and circulatory arrest) may change the indication for ultrafiltration during CPB.
Clinical Pictures
Classification and Diagnosis
Historically, the diagnosis of AKI was based on a rising serum creatinine (SCr) or a reduction in the urine output. However, these two parameters are delayed and unreliable measure in the acute setting (Bellomo et al. 2004a): age, gender, lean muscle mass, muscle metabolism and hydration status influence SCr, making its plasma levels variable. Moreover, a reduction of about 50 % of renal function is needed to measure a modification of SCr. At lower GFR rates, the amount of tubular Cr secretion results in overestimation of renal function. Finally, during acute changes in GFR, SCr does not accurately depict kidney function until steady-state equilibrium has been reached, which may require several days. Nevertheless, nowadays the absolute value of or a change in SCr remains the most widely used method for the diagnosis of AKI; in fact, there are more than 30 definitions of AKI in the current literature based on it. This lack of standardized definition has resulted in the reported incidence of AKI ranging from 1 to 25 % and a mortality ranging from 7 to 80 %, if aetiology is based on a primary renal disease or if it is part of a multiorgan dysfunction syndrome (MODS). We are given below of the most commonly used definition in the literature (Table 40.2).
Table 40.2
Definition of AKI by AKIN classification, RIFLE and pRIFLE scoring system
AKIN | RIFLE | pRIFLE | |||||
---|---|---|---|---|---|---|---|
Stage | SCr | Class | Urine output | SCr or GFR | Class | Urine output | eCrCl |
I | Increase > 0.3 mg/dL or >150–200 % from baseline | Risk | <0.5 mL/kg/h × 8 h | SCr increase 150 % or GFR decrease by 25 % from baseline | Risk | <0.5 mL/kg/h × 8 h | Decrease by 25 % |
II | Increase > 200–300 % from baseline | Injury | <0.5 mL/kg/h × 16 h | SCr increase 200 % or GFR decrease by 50 % from baseline | Injury | <0.5 mL/kg/h × 16 h | Decrease by 50 % |
III | Increase > 300 % from baseline or SCr > 4 mg/dL with an acute raise at least 0.5 mg/dL | Fail | <0.3 mL/kg/h × 24 h or Anuric × 12 h | SCr increase 300 % or SCr > 4 mg/dL with an acute increase of 0.5 mg/dL or GFR decrease by 75 % from baseline | Fail | <0.3 mL/kg/h × 24 h or anuric × 12 h | Decrease by 75 % or < 35 mL/min/1.73 m2 |
Loss | Failure > 4 weeks | Loss | Failure > 4 weeks | ||||
ESRD | Failure > 3 mouths | ESRD | Failure > 3 mouths |
RIFLE Classification System
In 2004 an interdisciplinary collaboration, the Acute Dialysis Quality Initiative (www.adqi.net) group, has defined the range of acute renal dysfunction using the so-called RIFLE classification system. It classifies three grades of increasing severity of acute renal dysfunction and two outcomes (Bellomo et al. 2004b):
Risk (R) – 1.5-fold increases in SCr, greater than 25 % decrease in GFR, or urine output of less than 0.5 ml/kg/h for 6 h
Injury (I) – twofold increase in SCr, greater than 50 % decrease in GFR, or urine output of less than 0.5 ml/kg/h for 12 h
Failure (F) – threefold increase in Scr (>4 mg/dl), greater than 75 % decrease in GFR, or urine output of less than 0.3 ml/kg/h for 24 h or anuria for 12 h
Loss (L) – persistent acute renal failure defined as the need for renal replacement therapy for greater than 4 weeks
End-stage renal disease (E) – need for renal replacement therapy for more than 3 months (Bellomo et al. 2004b)
The three severity grades are defined on the basis of the changes in SCr and/or GFR from baseline value and/or urine output where the worst of each criterion is used. The two outcome criteria, loss and end-stage renal disease, are defined by the duration of loss of kidney function. There is a reasonable correlation between stage of AKI based on RIFLE criteria and mortality. The criteria have been validated as predictive of mortality in studies on different adult patient populations, including cardiac surgical patients (Ricci et al. 2008a).
pRIFLE Classification System
In 2007, a modified paediatric version of the RIFLE classification system, pRIFLE, was proposed to classify AKI in paediatrics, and then it has also been developed and validated (Akcan-Arikan et al. 1997). It uses the same stages of acute kidney injury as the RIFLE criteria, but the SCr, GFR and urine output are inferior. pRIFLE criteria have been found to be predictive of outcomes, specifically mortality (Plotz et al. 2008), and it has been validated by various single-centre studies.
AKIN Classification System
In 2007, the Acute Kidney Injury Network (AKIN) developed a later version of the RIFLE classification: the new system classifies AKI into stages I–III on the basis of small (>/0.3 mg/dL) increases in serum creatinine levels or oliguria in 48 h (Mehta et al. 2007). Instead of RIFLE classification, the AKIN introduce some modifications:
AKI is defined by the decrease of renal function in about 48 h, defined as an increase in absolute SCr of at least 0.3 mg/dL, or defined by a percentage increase in SCr ≥50 % (1.5× first value) or by a decrease in the urine output (documented oliguria <0.5 mL/kg/h for more than 6 h); stage 1 corresponds to the risk class, but it also considers an absolute increase in SCr ≥0.3 mg/dL.
Stages 2 and 3 define the injury and failure classes, respectively; stage 3 also considers patients needing renal replacement therapy, independently of the stage (defined by SCr and/or UO).
A correct state of hydration and the exclusion of renal obstruction are necessary for the diagnosis of AKI.
The AKIN classification only does not consider either the GFR or baseline SCr; it requires at least two values of SCr obtained within a period of 48 h.
The two outcome classes (loss of kidney function and end-stage kidney disease) were removed from the classification.
Difference Between RIFLE, pRIFLE and AKIN Classification
The AKIN classification allows only the identification of more AKI patients, despite not exhibiting a better prognostic acuity in terms of in-hospital mortality. Moreover, it theoretically improves the RIFLE criteria sensitivity and specificity, although the advantages of the RIFLE modifications have not been proven (Lopes et al. 2008; Conlon et al. 1994).
The Multi-societal Database Committee for Pediatric and Congenital Heart Disease Classification
In 2008, the Multi-societal Database Committee for Pediatric and Congenital Heart Disease developed consensus definitions used by the STS Congenital Heart Surgery Database – the largest database on paediatric and congenital cardiac operations in the world – for renal dysfunction and renal failure requiring dialysis (Welke et al. 2008). Renal dysfunction was defined as oliguria with sustained urine output < 0.5 cc/kg per h for 24 h and/or a rise in creatinine > 1.5 times upper limits of normal for age, without the need for dialysis (including peritoneal dialysis and/or haemodialysis) or haemofiltration. Renal failure requiring dialysis was defined as oliguria with sustained urine output < 0.5 cc/kg per h for 24 h and/or a rise in creatinine > 1.5 times upper limits of normal for age, with the need for dialysis (including peritoneal dialysis and/or haemodialysis) or haemofiltration (Welke et al. 2008).
Lab Findings: New Biomarkers
Novel biomarkers for AKI have been developed in recent years, with potentially high sensitivity and specificity. These include plasma biomarkers (NGAL and cystatin C) and urine biomarkers (NGAL, IL-18, KIM-1 and L-FABP). Measurement should be technically easy with good reproducibility. So, it should be easier determining the timing of the initial insult, even in the absence of typical clinical signs, assessing the duration of AKI and correlating with both prognosis and response to treatment. Based on the differential expression of the biomarkers, it is also likely that the AKI panels will help distinguish between the various types and aetiologies of AKI and should enable early intervention.
Among the new markers, urine NGAL, IL-18 and serum cystatin C appear to perform best for the early diagnosis of AKI.
Neutrophil Gelatinase-Associated Lipocalin (NGAL)
Human NGAL is a small protein (around 25 KDalton) normally bound to gelatinase from neutrophils, involved in innate immunity. It is also present at very low levels, in several human tissues such as kidney, lungs, stomach and colon. Many studies found that in case of acute kidney injury, after an ischemic or nephrotoxic injury, NGAL gene is upregulated and the protein is secreted very quickly into the blood (Mishra et al. 2003). Because its small dimension, it is rapidly excreted, so it is easily and early detected in the urine. In patients who developed AKI, urinary NGAL levels increase within 2 h and peak at 6 h. These features have led many authors to consider NGAL as a biomarker of ischemic AKI (Mishra et al. 2005; Haase-Fielitz et al. 2009). However, it was found that NGAL is most sensitive and specific in relatively uncomplicated patients with AKI (Haase et al. 2009) and also its dosage can be affected by several variables, such as pre-existing renal disease and systemic or urinary tract infections (Picca et al. 1995).
Interleukin 18
IL-18 is a proinflammatory cytokine involved in cell-mediated immunity following infection with microbial products such as lipopolysaccharide. It was found that urinary level of IL-18 increases in ischemic AKI, but it is not influenced by nephrotoxins, chronic renal diseases or urinary tract infections. In cardiac surgery patients who developed AKI, urinary IL-18 was induced within 6 h and peaked at 12 h after CPB. So it can be used as marker of injured tubules. Urinary levels of NGAL and IL-18 have been proven to represent early, predictive, sequential AKI biomarkers in children undergoing cardiopulmonary bypass (Parikh et al. 2004).
Cystatin C
Cystatin C is a small protein normally produced by nucleated cells. It is a potent inhibitor of lysosomal proteinases and probably the most important cysteine proteinases inhibitor. It is removed from the bloodstream by glomerular filtration, and it is reabsorbed and catabolized but is not secreted by the tubules. If kidney function and GFR decline, the blood levels of cystatin C rise. Since the blood levels of cystatin C are not influenced by age, gender or muscle mass, it was found that serum levels of cystatin C are a more precise test of kidney function than SCr in patients with chronic kidney disease (Dharnidharka et al. 2002). Some studies showed that cystatin C can be considered a good predictor for renal replacement therapy need (Herget-Rosenthal et al. 2004a) and it might be predictive of AKI in intensive care setting (Herget-Rosenthal et al. 2004b). In a recent study in paediatric post-CPB patients, cystatin C levels at 12 h after CPB were strong independent predictors of AKI (Krawczeski et al. 2010).
The importance of the new biomarkers for AKI has been recently shown by Haase et al., confirming that an increase in the levels of these molecules is strongly related to an increased length of hospital stay, even in patients with normal creatinine value (Haase et al. 2011).
Treatment Course
Fluid Management
During the perioperative period, patients with chronic heart decompensation require a great amount of fluid. As a consequence, fluid management became of utmost importance: the volume needed should be administered to maintain perfusion, but FO should be avoided. Fluid intake calculation should take into account all sources of fluid, including intravenous crystalloid, parental nutrition, drug infusion, fluid bolus. Fluid management is a challenging task: in the past, a positive fluid balance was associated with a clinical benefit; nowadays, there are increasing evidences that positive fluid balances in the order of 5–10 % of body weight are associated with worsening organ dysfunction in the critically ill and with worse postoperative outcomes after surgery. In clinical practice, fluid management after congenital heart disease surgery is more complicated considering the risk of prerenal AKI in case of excessive fluid restriction and the risk of kidney damages related to fluid and sodium overload and the effect of acidosis and hyperchloraemia on renal flow. As a consequence, the amount and the kind of infused fluid play a crucial role in determining or worsening a renal dysfunction.
Fluid Overload
Fluid overload, deriving from the inability or impossibility to optimize endovascular filling, has been clearly identified as an independent risk of mortality, length of mechanical ventilation and hospital stay (Ricci et al. 2011a). Particularly, because of an increased TBW amount and the highest possibility of interstitial fluid spill over, smallest patients and newborns are the most delicate patients, especially when inflammatory conditions are present, such as post CPB. So, the prevailing consensus is to avoid a hypervolaemic state in the immediate postoperative period as an FO is considered a risk factor for multiorgan dysfunction. Different factors contribute to the development of FO. CPB leads to haemodilution and increased capillary permeability, both of which promote extravasation of fluid into the ECS. Moreover, postoperative administration of fluid and blood product worsens the third-space accumulation of water. Subsequently, increasing oedema leads to the raising of the intra-abdominal pressure, decreasing renal perfusion. When combined with postoperative myocardial dysfunction, there is also a stimulus FO via the RAAS. Given the acute nature of CPB-mediated kidney injury and the observation that most patients have normal renal function prior to surgery, these patients may be ideal candidates for aggressive postoperative goal-directed protocols aimed at avoiding FO and optimizing tissue perfusion.
Goal-Directed Fluid Therapy
Adequate fluid resuscitation is essential to restore cardiac output, systemic blood pressure and renal perfusion in patients with shock secondary to low cardiac output, but fluid responsiveness of cardiac output is dependent both on the volume of the central venous reservoirs and venous tone. When managing fluid resuscitation, the best strategy may be to ensure a sufficient preload to generate adequate cardiac output rather than simply responding to hypotension. Goal-directed therapy (GDT) allows physicians to the treatment of hypotension, including volume expansion with boluses of crystalloid or colloid, or the use of inotropes, only to patients who need them, in order to assure sufficient DO2 to fulfil the metabolic requirement of the particular patients. This approach may help to determine when fluid resuscitation can safely be stopped, avoiding FO (Agrò and Vennari 2014). Defined haemodynamic variables are necessary to evaluate volume status and to test fluid responsiveness. Filling pressure, central venous pressure (CVP), pulmonary capillary wedge pressure (PCWP) and mean arterial pressure (MAP) are the most commonly used and known parameters to evaluate fluid replacement in cardiosurgical ICU (Kastrup et al. 2007). Despite being easily achieved and their values simply interpreted, they have limited predictive value as indicators of fluid responsiveness due to the different underlying cardiac compliance and value competence (Osman et al. 2007). Many studies have shown that CVP does not adequately reflect preload and fails to predict fluid responsiveness (Agrò and Vennari 2014; Kastrup et al. 2007). In one study PCWP was found to adequately predict fluid responsiveness in 19 patients who have undergone CABG (Bennett-Guerrero et al. 2002). Other studies found a significant CVP and PCWP increase after a fluid challenge, but they do not correlate to an increase in stroke volume (Wiesenack et al. 2001). Moreover, pressure parameters are altered by intra-abdominal pressure variation, modification of cardiac compliance, pulmonary resistance, and cardiac pathologies (Marik et al. 2008). As a result they are not very reliable and useful in cardiac surgery patient who present at less one of these conditions. More recently, the use of respiratory variation of arterial pressure, such as pulse pressure variation (PPV), stroke volume variation (SVV) and continuous cardiac index (CI) to predict fluid responsiveness, has shown some interesting data in both operating room and intensive care units (Teboul and Monnet 2009). Both high PPV and SVV are indicators of hypovolaemia, indicate fluid responsiveness and correlate to the CI increase after fluid challenge administration (Habicher et al. 2011). This evidence was confirmed in a study on off-pump CABG patients, in which both SVV and PPV strongly correlated to CI improvement after a fluid challenge, with respect to filling pressure (Habicher et al. 2011). As a consequence, dynamic parameters such as PPV and SVV are able to adequately distinguish fluid responder and fluid nonresponder patients and are suitable to guide fluid management in the perioperative period of cardiac surgery, with respect to filling pressure. Unfortunately, these dynamic indices, robust and reliable under specific conditions (mechanical ventilation, closed chest, sinus rhythm, low tidal volume ventilation, absence of right ventricular failure), have not been validated during open-chest settings.
The esophageal Doppler (ED) estimations might be more reliable, even if biased by the need of a learning curve. It allows measurement such as left ventricular end-diastolic area (LVEDA) and the blood velocity at the level of the descending aorta. The flow time in the descending aorta, corrected for HR (FTc normally 330–360 ms), corresponds to the SV. At lower velocities, hypovolaemia should be suspected. FTc correlates with LVEDA (Agrò and Vennari 2014). ED requires shorter operator training than other systems and does not require calibration, but it is difficult to use in awake patients and in a prolonged monitoring, and finally its results may be operator dependent. GDT using ED was shown to improve patient outcomes (Agrò and Vennari 2014).
In the last years, new devices, assessing dynamic and volumetric parameters, have been developed. They are PICCO system and PiCCO2, PULSION Medical Systems, Munich, Germany; FloTrac, Edwards Lifesciences; and LiDCOrapid, LiDCO, London, UK. These devices use trans-pulmonary thermodilution and/or pulse wave analysis. When the methodologies are used in combination, the validity of pulse pressure analysis depends on periodic recalibration through thermodilution (Agrò and Vennari 2014; Reuter et al. 2010). These systems require invasive arterial lines and a central venous catheter, but they are less invasive than PAC. All these systems have to be compared to the Swan–Ganz or pulmonary artery catheter (PAC), which remains the gold standard, despite its limits. In fact, many clinical trials showed that PAC is not suitable for GDT in the routine perioperative setting. Its use is further discouraged by the invasiveness of the procedure, which exposes patients to complications. In addition, PAC cannot be used without adequate training and experience. Finally, its performance mainly refers to filling pressure values (PVC, PCWP), which have been found to be not so effective in clinical practice. Consequently, its fame in the literature and in clinics has decreased over the years (Agrò and Vennari 2014). Low cardiac output should be managed with a multimodal monitoring and treatment tailored to the single patient and clinical picture trying to obtain the best balance between fluids, inotropes and vasopressors during the whole intra- and postoperative phase.
The maintaining of an adequate cardiac output with no FO is the first goal of perioperative fluid management in cardiac heart surgery, avoiding primary, secondary and iatrogenic renal dysfunction.
Type of Fluid Solution
In 2001, a survey of paediatric anaesthetists suggested that the choice of fluids for plasma volume expansion in infants and children varied by geographical location, with semi-synthetic colloids commonly used and albumin mainly used for neonates (Soderlind et al. 2001). Recently, the evidence about which fluid to use are changed, suggesting caution regarding the use of albumin, the use of semi-synthetic colloids and excessive volumes of intravenous crystalloid in perioperative or critically ill patient (Myburgh and Mythen 2013). At the state of the art, crystalloids are suggested for continuous losses (perspiratio insensibilis and urinary output), while colloids are suggested for temporary losses (IVS loss, such as due to haemorrhage) (Agrò et al. 2013a).
Colloids
Colloids are distributed in the IVS, with a larger increase in plasma volume because they contain oncotic particles. They have a longer duration of action, with smaller volumes needed for a specific target volume expansion than crystalloids (Agrò et al. 2013a). If endothelial permeability is intact, colloids are retained in the IVS, with a subsequent increase of the plasma oncotic pressure and the diffusion of fluids from the ISS to the IVS (Agrò and Vennari 2014). Colloids have a ‘contest volume effect’: in hypovolaemic patients, they have a volume effect > 90 % of the volume infused; in normovolaemic patients, two-thirds of the infused volume shifts to the ISS within minutes. Consequently, they should be used only in hypovolaemia, even when there is capillary membrane damage. In fact, in this case, hypovolaemia is connected to the shift into the ISS of protein-rich fluids, with a plasma COP reduction. Colloids that are able to increase COP are needed: their use may reduce ISS overload.
In 2006, Verheij et al. (2006a) showed that following cardiac surgery, volume expansion and cardiac output were significantly higher after colloid infusion than after the administration of crystalloids. He found colloids were approximately five times as efficient in expanding the IVS volume with respect to saline 0.9 %. Ley et al. (1990) compared fluid replacement with crystalloids or colloids in patients undergoing coronary artery bypass or valve substitution. Patients treated with HES showed a reduced length of ICU stay than patients treated with normal saline solution. In addition, they required less fluid infusion after surgery and showed better haemodynamic performance than the crystalloid group (Agrò et al. 2013a).
Despite this evidence, colloids have been associated with coagulopathy, and platelet dysfunction, predisposing cardiac surgery patients to postoperative bleeding (in particular when high MW molecules and CPB are involved) and to anaphylaxis (especially gelatins), may cause tubular damage with renal dysfunction (Agrò et al. 2013a). All colloids can induce kidney injury. The anatomic feature of colloid-induced renal damage is an osmotic nephrosis-like lesion. The most likely mechanism of renal dysfunction is a tubular obstruction caused by hyperoncotic urine formation with the storage of colloidal molecules filtered by the glomeruli. This mechanism is further impaired by a condition of dehydration. Another suggested mechanism is an increase in plasma oncotic pressure, with secondary renal macromolecules accumulation. Adequate hydration using crystalloids may prevent this injury (Agrò et al. 2013b). The proposed risk factors for colloid-related kidney dysfunction are age (older patients have a higher risk), hypovolaemia, previous kidney alterations (chronic or acute injury due to other causes) and other comorbidities (such as diabetes and others conditions causing direct or indirect renal alterations). Other risk factors are the type of colloid administered (higher MMW and MS) and the total amount infused per kg of body weight (Agrò et al. 2013b). Clinical evidences of the renal effects of colloid use (especially hydroxyethyl starches) are not uniform, and there is still intense debate as to whether there is truly a critical creatinine level for their administration. Up to some years ago, the use of low MMW and low MS hydroxyethyl starches (HES) was thought to be relatively safe on renal function with respect to other colloids. More recently many issues have been relieved by literature. Initially the administration of the newest-generation HES was suggested to reduce the risk of short-term and long-term renal injury (Agrò et al. 2013b; Mitra and Khandelwal 2009). In a study on brain-dead kidney donors, Blasco et al. (2008) compared HES 130/0.4 and HES 200/0.62. At 1 month and 1 year post-administration, they found better effects on renal function (lower serum creatinine) with HES 130/0.4 than with HES 200/0.62 (Feng et al. 2006). The use of fourth-generation HES seems to cause much less harm than older-generation HES, even in patients with previous renal impairment. The infusion of 500 mL of HES 6 %/130/0.4 did not cause any kidney damage in volunteers showing mild-to-severe renal dysfunction (Agrò et al. 2013b; Jungheinrich et al. 2002). In a review comprising 34 studies (2607 patients), HES was compared with other fluids. According to other studies, the results evidenced an increased risk of acute renal dysfunction, of long-term renal damage and mortality with HES (even third- and fourth-generation HES), especially in patients with sepsis (Agrò et al. 2013b; Perner et al. 2012; Myburgh et al. 2012). On the basis of these evidences, HES use (included modern HES) has been restricted in Europe, with specific reference to patient with renal dysfunction or undergoing dialysis. Their use remains justified in case of severe hypovolaemic shock. According to the recent literature, the newest-generation HES seems to be the better colloidal solutions with respect to kidney oncotic damage while assuring an adequate volume replacement. However, the influence of HES on kidney function remains controversial, and large studies are still needed to evaluate the incidence of acute kidney injury with HES in patients without sepsis, directly applying the RIFLE criteria, by precisely measuring the GFR and urine output together with creatinine and NGAL (Young 2012; Agrò et al. 2013b). The need for studies with a specific subset of patients (i.e. cardiac surgery patients) is crucial in the perioperative management of population with a high risk of AKI, such as congenital heart disease patients, considering colloid use is largely diffused in the intra- and postoperative setting (i.e. CBP priming).
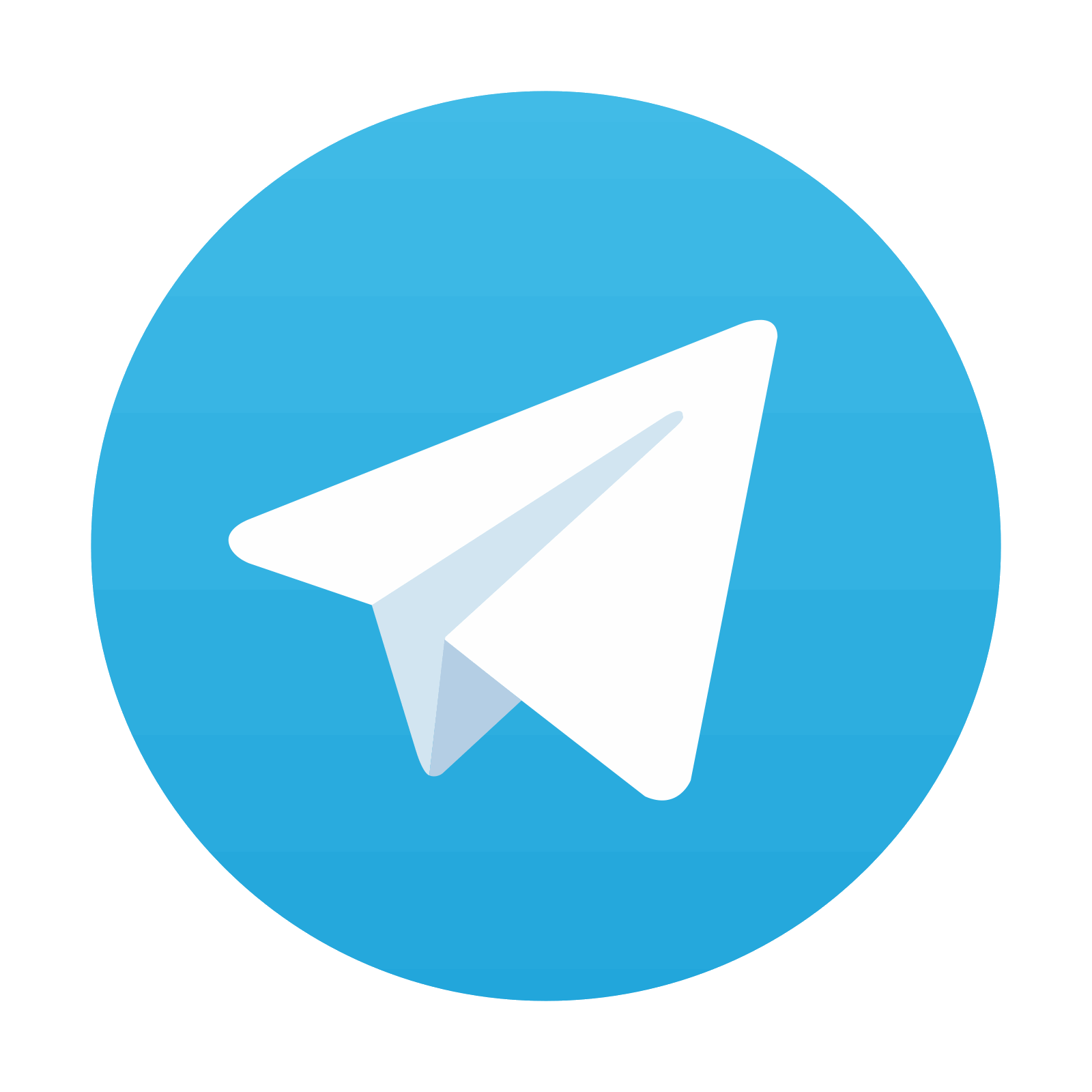
Stay updated, free articles. Join our Telegram channel
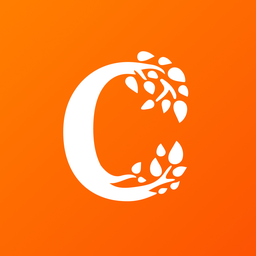
Full access? Get Clinical Tree
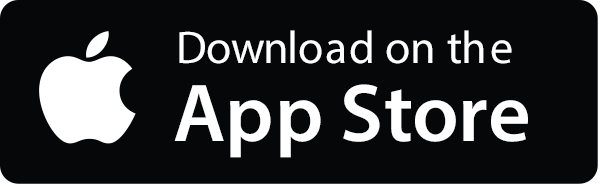
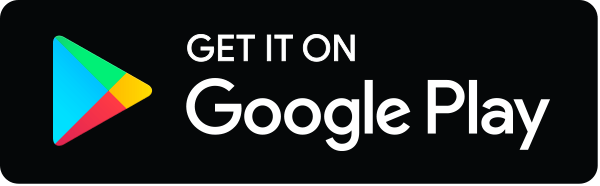
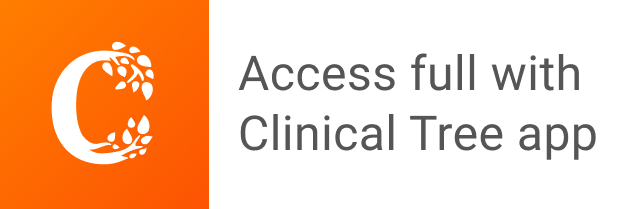