In this equation, R = the universal gas constant, T = absolute temperature, F = the Faraday constant, and X is the ion in question. As an example, the usual intracellular and extracellular concentration of potassium is 4.0 mM and 140 mM, respectively. Substituting these values into the Nernst equation gives the following values:
![$$ {E}_k=61\kern0.24em \ln \left[4\right]\;/\;\left[140\right]=-94\kern0.24em \mathrm{mV}. $$](/wp-content/uploads/2016/07/A107938_2_En_2_Chapter_Equb.gif)
The membrane potential at any given moment may be calculated if the corresponding instantaneous intra- and extracellular concentrations of the ions and the permeability of the respective ion channels are known. The Goldman–Hodgkin–Katz equation describes the membrane potential for any given set of ion concentrations [X] and permeability’s (P). For potassium [K], sodium [Na], and chloride [Cl], the equation is:
(The chloride ion concentration is inverted to account for its negative charge.) The Goldman–Hodgkin–Katz equation more closely approximates the cellular potential than the Nernst equation because it accounts for the permeability of the membrane for all active ions. This equation is used to calculate ion channel permeability from single channel experiments and then apply to computer models of single cells and cellular syncytia.
![$$ {V}_m=RT/F \ln \left\{{P}_{\mathrm{K}}{\left[{\mathrm{K}}^{+}\right]}_{\mathrm{o}}+{P}_{\mathrm{Na}}{\left[{\mathrm{Na}}^{+}\right]}_{\mathrm{o}}+{P}_{\mathrm{Cl}}{\left[{\mathrm{Cl}}^{+}\right]}_{\mathrm{i}}\right\}/\left\{{P}_{\mathrm{K}}{\left[{\mathrm{K}}^{+}\right]}_{\mathrm{i}}+{P}_{\mathrm{Na}}{\left[{\mathrm{Na}}^{+}\right]}_{\mathrm{i}}+{P}_{\mathrm{Cl}}{\left[{\mathrm{Cl}}^{+}\right]}_{\mathrm{o}}\right\}. $$](/wp-content/uploads/2016/07/A107938_2_En_2_Chapter_Equc.gif)
The transmembrane potential for the cardiac myocyte at baseline is defined as the resting membrane potential. The sarcolemma is nearly impermeable (highly resistant) to sodium and calcium ions while the conductance (conductance = 1/resistance) for potassium ions is high. As such, the resting membrane potential of most cardiac myocytes approaches the equilibrium potential for potassium, generally ranging from –80 to –90 mV.
The maintenance of the resting membrane potential is an active, energy-dependent process. The most important membrane proteins for establishing the resting membrane potential are the Na+/K+-ATPase (ion exchanger) and the potassium channel responsible for the inward rectifying potassium current (I K). The Na+/K+-ATPase is an electrogenic pump that exchanges three sodium ions from the inside of the cell for two potassium ions in the extracellular space, resulting in a net outward flow of positive charge.
Ion Channels
Ion channels are macromolecular proteins that span the sarcolemma and provide a low resistance pathway for ions to enter or exit the cell. The ion channels are selective for specific ions to pass down their electrochemical gradient. The ion channels have three general properties: (1) a central water-filled pore through which the ions pass; (2) a selectivity filter; and (3) a gating mechanism to open and close the channel. The channels may be classified not only by their selectivity for specific ions, but also by the stimulus that causes the channel to open. Channels may open in response to changes in the transmembrane potential (voltage gated), in response to activation with various ligands (messenger gated), in response to mechanical forces (stretch activated), and in response to changes in the metabolic state of the cell (ATP gated).
Sodium Channels
The sodium channels provide the pathway for the sodium current, the principal current responsible for cellular depolarization in atrial, ventricular, and Purkinje fibers. They are also present in skeletal muscle and neuronal cells which can be differentiated from cardiac sodium channels by their greater sensitivity to tetrodotoxin. The cardiac sodium channels are proteins composed of a large pore-forming α-subunit and two smaller regulatory β-subunits. The α-subunit consists of four homologous domains, each of which consists of six transmembrane segments, S1–S6 (Fig. 2.1), a motif that is consistent across the voltage-gated ion channels. The transmembrane segments are hydrophobic and have an alpha-helical conformation. The fourth transmembrane segment (S4) in each domain is highly charged with arginine and lysine residues located at every third position. The S4 segment acts as the voltage sensor for the channel with membrane depolarization causing an outward movement of all of the S4 domains leading to an opening of the transmembrane pore. The channel pore is formed by the S5 and S6 segments of each of the four domains in addition to the extracellular linker between S5 and S6. The transmembrane segments are linked by short loops, which alternate between intra- and extracellular. The extracellular linker loop between S5 and S6 is particularly long and curves back into the lipid bilayer to line the pore through which the ions pass. The four extracellular S5–S6 linker loops contribute to the selectivity of the channel.
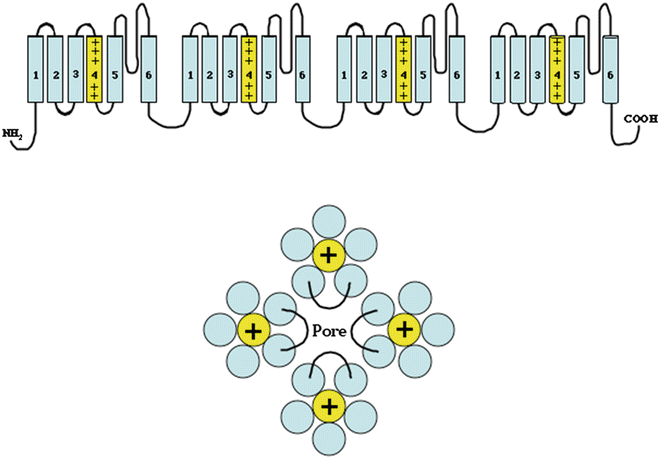
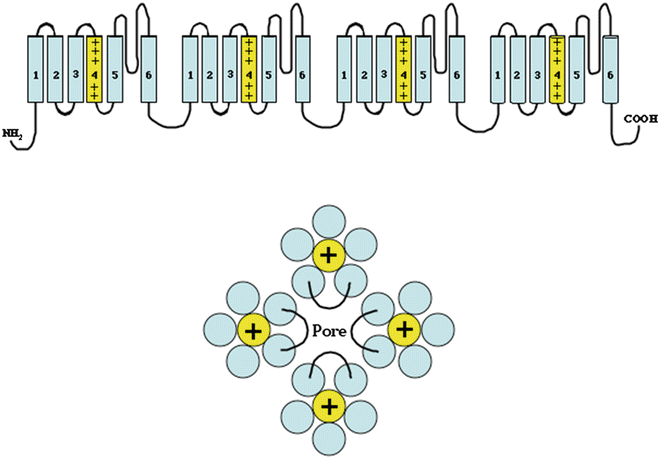
Fig. 2.1
Top: Drawing of a voltage-gated sodium channel. The channel is composed of four domains, each of which has six membrane-spanning hydrophobic helical segments. The fourth transmembrane segment is highly charged and acts as the voltage sensor for the channel. The linker segment between the fifth and sixth transmembrane segment in each domain bends back into the channel pore and is important in channel selectivity and gating. Bottom: This idealized drawing viewed from the extracellular surface demonstrates how the four domains organize to form a single pore with the S5–S6 linker segment of each domain contributing to the pore
The function of the β-subunit continues to be investigated. In addition to modulating channel-gating properties, the subunit serves as a cell adhesion molecule that interacts with the extracellular matrix serving an anchoring function. The β-subunits also regulate both the level of channel expression and the trafficking of the completed protein to the plasma membrane. In the last few years, genetic mutations leading to errors in ion channel trafficking have been linked to arrhythmogenesis. A mutation in the Nav1.5 gene (gene encoding the sodium channel) associated with Brugada Syndrome has been demonstrated to result from poor subunit trafficking. Interestingly, the class Ia antiarrhythmic drug quinidine’s efficacy has been linked, in part, to increased Na-channel endocytosis (the energy-using process by which cells absorb molecules by engulfing them).
The sodium channel opens rapidly in response to a depolarization in the membrane potential above a threshold value, reaching its maximal conductance within half a millisecond. After opening, the sodium current then rapidly dissipates, falling to almost zero within a few milliseconds. The inactivation of the sodium channel is the result of two separate processes, which may be differentiated based on their time constants. An initial rapid inactivation has a fast recovery constant and is, in part, caused by a conformational change in the intracellular linker between S3 and S4 that acts like a ball valve swinging into and occluding the pore-forming region. Rapid inactivation may occur without the channel opening, a process known as “closed state inactivation.” A slower, more stable inactivated state also exists and may last from hundreds of milliseconds to several seconds. The mechanism(s) underlying slow inactivation are not well understood but likely result from the linker sequences between S5 and S6 in each domain bending back into the pore of the channel and occluding it (Fig. 2.1).
The SCN5A gene located on chromosome 3 encodes the cardiac sodium channel, Nav 1.5. Several genetically mediated arrhythmias in humans resulting from cardiac sodium channel gene mutations have been identified (Chap. 18).
Potassium Channels
Potassium channels are major components in the establishment of the resting membrane potential (see above) and, as will be discussed, cardiac myocyte automaticity. These channels are more numerous and diverse than any other type of ion channel in the heart. In mammals, over 75 genes have been identified that code for potassium channels. The channels may be categorized by their (1) voltage, (2) time, and (3) molecular structure, dependent properties, as well as their response to pharmacological agents. Within the heart a tremendous amount of heterogeneity exists in the density and expression of the potassium channels. The varied expression level of potassium channels contributes to the variability of the action potential morphology in different regions of the heart including transmural differences within the ventricular myocardium. In addition to the natural variability in the expression of potassium channels, many disease processes such as congestive heart failure and persistent tachyarrhythmias, such as chronic atrial fibrillation, alter the density of these channels, as well as their functional properties, thereby leading to disruption of the normal electrical stability of the heart. This alteration in the density and function of these channels has been termed “electrical remodeling.”
Voltage-Gated Channels
Voltage-gated potassium channels are structurally very similar to the voltage-gated sodium channels. One major difference is that instead of the channels being composed of a single large α-subunit containing four domains, they are heteromultimeric complexes consisting of four α-subunits that form the channel pore and covalently attached regulatory β-subunits. The α-subunits are the equivalents of the separate domains of the sodium channel and are composed of six membrane-spanning segments (Fig. 2.2). The voltage sensor is contained in the S4 transmembrane segments that possess the same highly charged construct as in the sodium channel with alternating arginine and lysine in every third position. The mechanism supporting channel activation has not been as clearly delineated in potassium channels as in sodium channels. Identical to the sodium channel, the fifth and sixth transmembrane segments and the extracellular linker loop between S5 and S6 form the pore. While multiple subfamilies of potassium channel α-subunits exist, only closely related subfamilies of α-subunits are capable of co-assembling to form functioning channels.
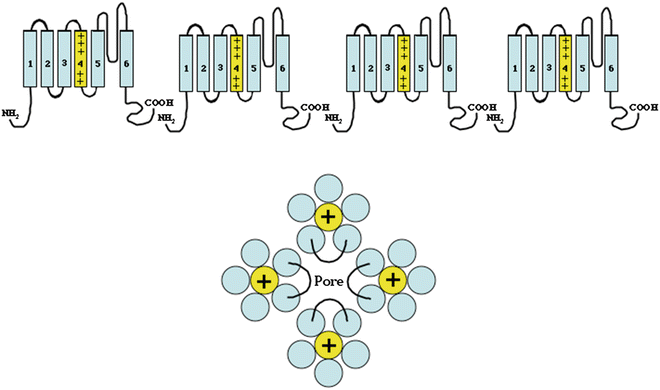
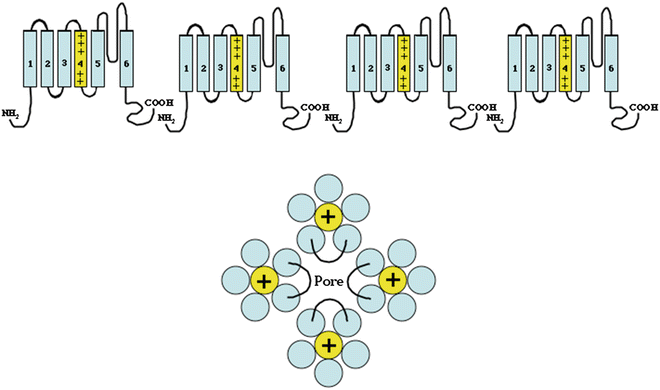
Fig. 2.2
Similar to the voltage-gated sodium channels, the voltage-gated potassium channels are composed of four domains (α-subunits) composed of six membrane-spanning segments. Unlike the sodium channels, the potassium channel domains are separate subunits that co-assemble to form a functional channel (compared with the sodium channel, which is a single large α-subunit composed of four domains. These four α-subunits assemble to form a single pore (structurally similar to the sodium channel, Fig. 2.1) with the S5–S6 linker from all α-subunits contributing to the pore. Similar to the voltage-gated sodium channel, the S4 subunit is also highly charged and serves as the voltage sensor leading to channel opening and closing
Inwardly Rectifying Channels
The inwardly rectifying channels are structurally distinct from the voltage-gated channels. As opposed to the four membrane-spanning subunits in voltage-gated channels, the inwardly rectifying channels have two membrane-spanning subunits (M1 and M2). The association of four subunits forms a pore (Fig. 2.3). The ATP-gated channel (I K-ATP) is more complex with the four pore-forming subunits co-assembling with four sulfonylurea receptors to form a functional channel. Inward rectification occurs via gating of the channels by magnesium and polyamines (spermine, spermidine, etc.) that block the inner opening of the pore.
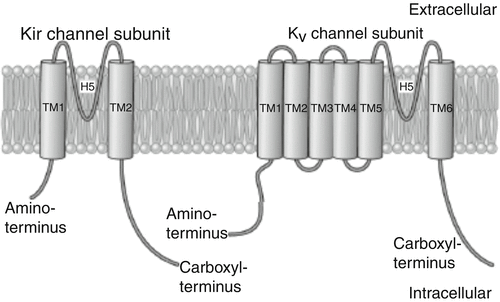
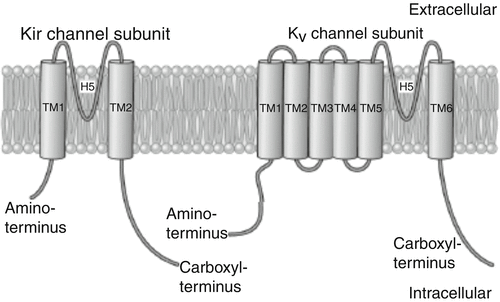
Fig. 2.3
Basic structure and Kir channel phylogenetic tree. A: primary structure of the Kir channel subunit (left). Each Kir subunit contains two transmembrane (TM1 and TM2) regions, a pore-forming (H5) loop, and cytosolic NH2 and COOH termini. As a comparison, the structure of voltage-gated K+ (K v) channel subunit, which possesses six transmembrane (TM1–TM6) regions, is shown on the right
I K1 is the dominant resting conductance current in the heart, setting the resting membrane potential in atrial, ventricular, and Purkinje cells. The heterogeneous density of channel distribution is greater in the ventricles relative to the atrium, but relatively sparse in nodal cells. I K1 has been demonstrated to inactivate at sustained depolarized potentials, such as during the plateau phase of the action potential.
Acetylcholine, which is released from the cardiac parasympathetic nerves, acts on type 2 muscarinic receptors to open I K-Ach channels via a G-protein-dependent mechanism. The channels are localized primarily in nodal cells and atrial myocytes. The presence of I K-Ach channels in the ventricle has been identified, although the sensitivity to ACh is less than in the nodal cells and atrial myocytes. Activation of the channels causes hyperpolarization of nodal cells and a slowing of the rate of spontaneous depolarization and shortening of the action potential in atrial and ventricular myocytes.
Molecularly Active Channels
I K-ATP is tonically inhibited by physiological concentrations of intracellular ATP. During periods of metabolic stress, when the ATP level decreases and the ATP/ADP ratio is altered, the inhibition on the channel is lost and the channel opens, providing a large conductance repolarizing current (outward movement of K+). Two molecularly distinct populations of I K-ATP have been described in the heart: one existing in the sarcolemma and the other in the inner mitochondrial membrane. IK-ATP, and in particular, the mitochondrial channel, has been demonstrated to be important in ischemic preconditioning.
Calcium Channels
Calcium channels share the same basic structural motif as the voltage-gated potassium channels and the voltage-gated sodium channels. The channels are composed of a single large pore-forming α-subunit, with two regulatory subunits (β, α2/δ). The α-subunit is similar in structure to that of the sodium channel, consisting of a single large protein with four domains composed of six membrane-spanning segments (Fig. 2.4). The voltage sensor is also localized on S4 and the pore is composed of S5, S6, and the S5–S6 linker of each of the four domains. However, the pore-forming loop between S5 and S6 is significantly different between calcium and sodium channels. The calcium channel has several calcium-binding sites on the pore-forming loop and the presence of calcium at these sites blocks sodium from entering the channel pore. When these sites are devoid of calcium, the channel passes sodium ions freely.
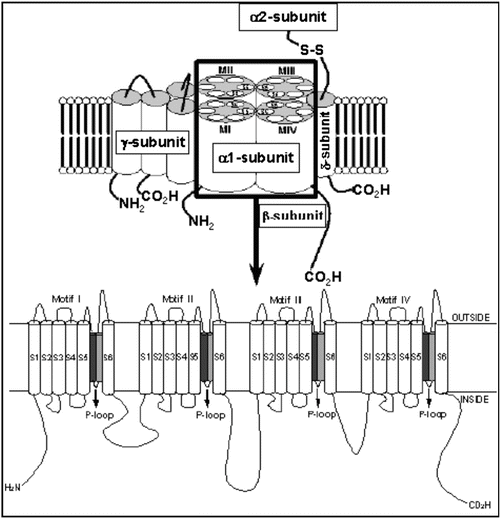
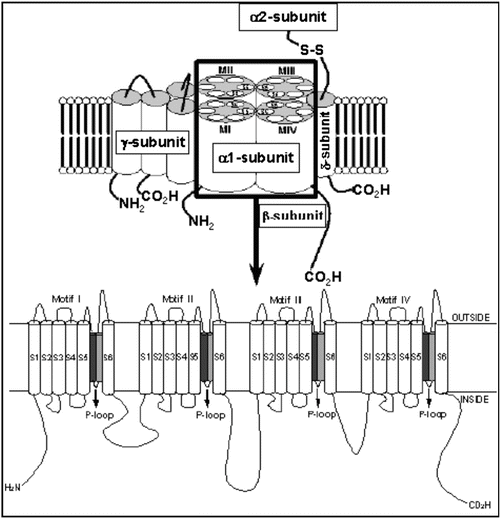
Fig. 2.4
L (transient) type calcium channel (voltage-gated). Molecular structure of the VGCC complex with zoom of the proposed transmembrane arrangement of the VGCC α 1-subunit. The dark and light gray cylinders are the SS1 and SS2 segments, respectively
There are two main types of calcium channels embedded in the sarcolemma which are differentiated by their conductance, activation/inactivation, and their response to pharmacological agents. The L-type calcium channel (I Ca,L—long acting) compared with the T-type channel (I Ca,T—transient channel) activates at more positive membrane potentials, inactivates more slowly, and has a larger single channel conductance. L-type calcium channel blocker drugs are used as cardiac antiarrhythmics or antihypertensives, depending on whether the drugs have higher affinity for the heart (the phenylalkylamines, like verapamil), or for the vessels (the dihydropyridines, like nifedipine). I Ca,L is expressed abundantly in all myocytes responsible for bringing calcium into working myocytes and leading to calcium-dependent calcium release from the sarcoplasmic reticulum. The expression of I Ca,T is more heterogeneous, being most prominent in nodal cells.
Chloride Channels
Far less is known about the structure and function of cardiac channels that carry anions. There are at least three distinct chloride channels in the heart. The first is the cardiac isoform of the cystic fibrosis transmembrane conductance regulator (CFTR), which is regulated by cAMP and protein kinase A. The second is a calcium-activated channel that participates in early repolarization, and the third is a swelling activated channel. To date, no role for abnormal function of chloride channels has been found for arrhythmia formation, and the channels are not targeted by any of the currently available antiarrhythmic drugs despite having a clear effect on action potential duration.
Gap Junctions
Gap junctions are tightly packed protein channels that provide a low resistance connection between adjacent cells, allowing the intercellular passage of ions and small molecules. These channels allow the rapid spread of the electrical signal from cell to cell. They are produced by the non-covalent interaction of two hemi-channels (connexins) that are embedded in the plasma membranes of adjacent cells. A connexin is formed by the association of six connexin subunits, each of which has four transmembrane-spanning domains and two extracellular loops. There are greater than 12 types of connexins expressed in myocardium. The transmembrane domains and extracellular loops in all of the connexins are highly preserved while the intracellular loop between the second and third domain and the carboxy-terminus are highly variable. The differences in the intracellular portions of the connexins account for the difference in molecular weight and physiological properties such as the junction conductance, pH dependence, voltage dependence, and selectivity.
The connexins are named by their molecular weight, e.g., Cx40 weighs 40 kDa. The distribution of connexins within the heart is variable and changes between embryonic development and the adult heart. Connexins 40 and 43 predominate in the atria, 43 in the ventricle, and 40 and 45 in the specialized conduction tissue of the sinus node and His/Purkinje system. Heterogeneous placement in the heart as well as its location along the axis of the cardiomyocyte at the desmosome also facilitates sequential depolarization of the cell and propagation of the action potential. The embryologic differential expression of the connexins has been implicated as a possible etiology for some forms of congenital heart disease.
The Action Potential
The action potential is a time-dependent transcription of the change in voltage across the sarcolemma (cell membrane). It is divided into five phases, delineated by the dominant membrane conductance (Fig. 2.6). The action potential of fast response and slow response cells differ and will be addressed separately below:
Phase 0
The sodium current is the principal current responsible for cellular depolarization in atrial, ventricular, and Purkinje fibers. The rapid flow of ions through the sodium channel permits rapid depolarization of the sarcolemma as well as rapid conduction of the electrical signal between cells. Sodium channels are closed at normal hyperpolarized resting membrane potentials. When stimulated by membrane depolarization, they open allowing the rapid influx of sodium ions, which changes the membrane potential from −90 mV towards the equilibrium potential for sodium of +40 mV. The channel then inactivates rapidly over a few milliseconds in a time-dependent fashion—that is, even in the face of a sustained depolarized membrane potential, the channel will close after a short time.
This phase includes the rapid depolarization of the membrane. At rest, as discussed earlier, the membrane of a fast response cell is permeable almost exclusively to potassium. This drives the resting membrane potential towards the equilibrium potential of potassium (−94 mV). If the membrane potential is depolarized beyond a set threshold value, the voltage-gated sodium channels open and the membrane’s dominant conductance changes to sodium. The membrane potential therefore moves towards the equilibrium potential of sodium (I Na ~ +40 mV). The stimulus that generates the action potential elicits an all-or-nothing response. If the stimulus is subthreshold, the membrane is partially depolarized and then quickly returns to the resting potential. If the stimulus is of sufficient intensity to raise the membrane potential above the threshold level, a maximal response is elicited and an action potential is generated. If the threshold potential is reached, phase 0 of the action potential is not altered by the greater intensity of the stimulus (i.e., a stimulus of greater intensity does not result in an increase in V max). The number of available sodium channels is dependent on the resting membrane potential of the cell and determines the V max. If the resting membrane potential is depolarized (less negative) relative to the normal value (i.e., ~−90 mV), fewer sodium channels are available to be recruited to participate in the action potential due to a greater number of sodium channels in inactivated states and the V max is lower. Clinically, this may occur during myocardial ischemia or other instances of stress. Sodium channel blocking antiarrhythmic drugs produce a decrease in dV/dt max (upstroke velocity of phase 0, Fig. 2.5) and result in a prolonged phase 0.
< div class='tao-gold-member'>
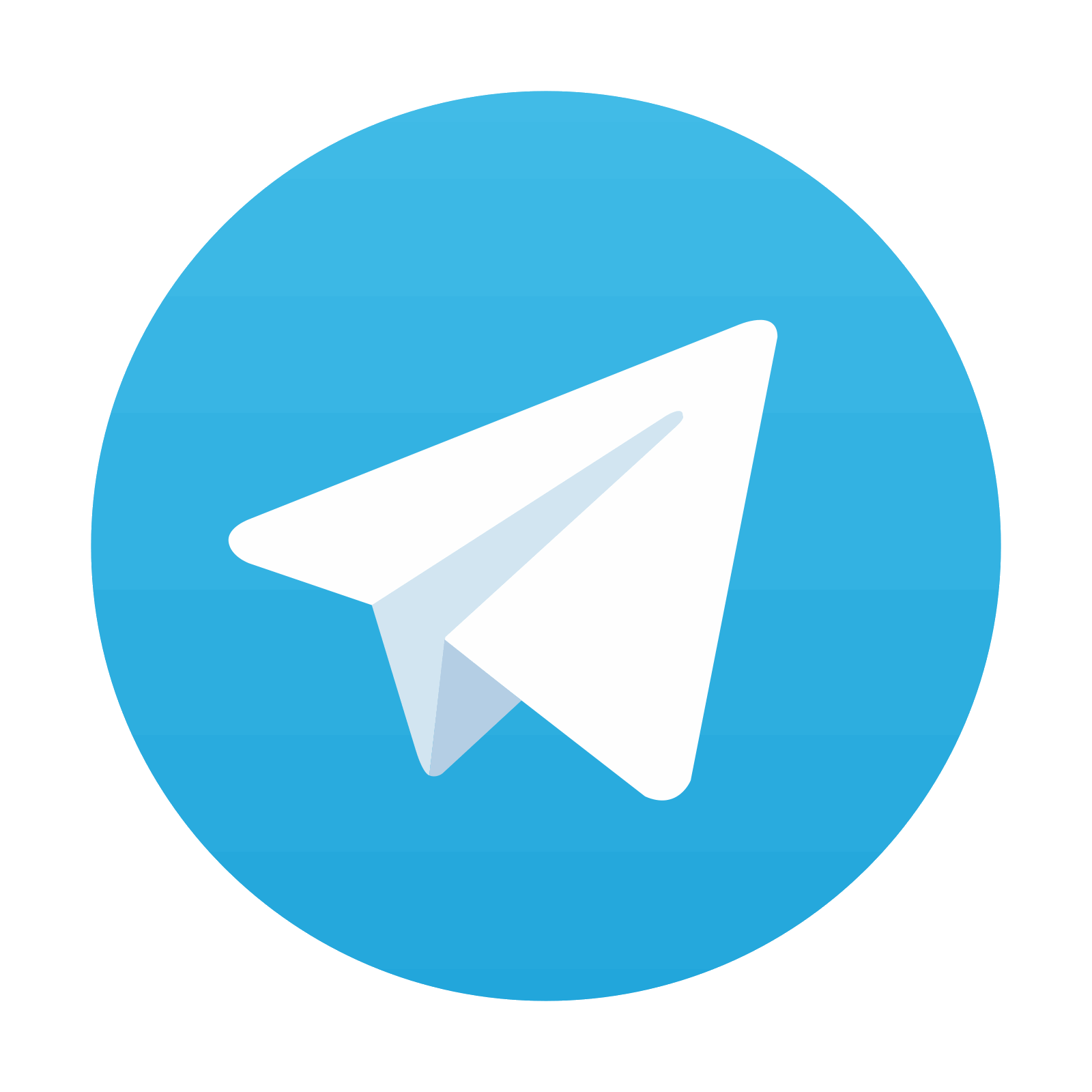
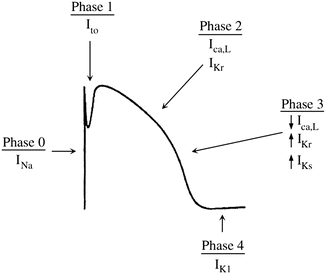
Only gold members can continue reading. Log In or Register a > to continue
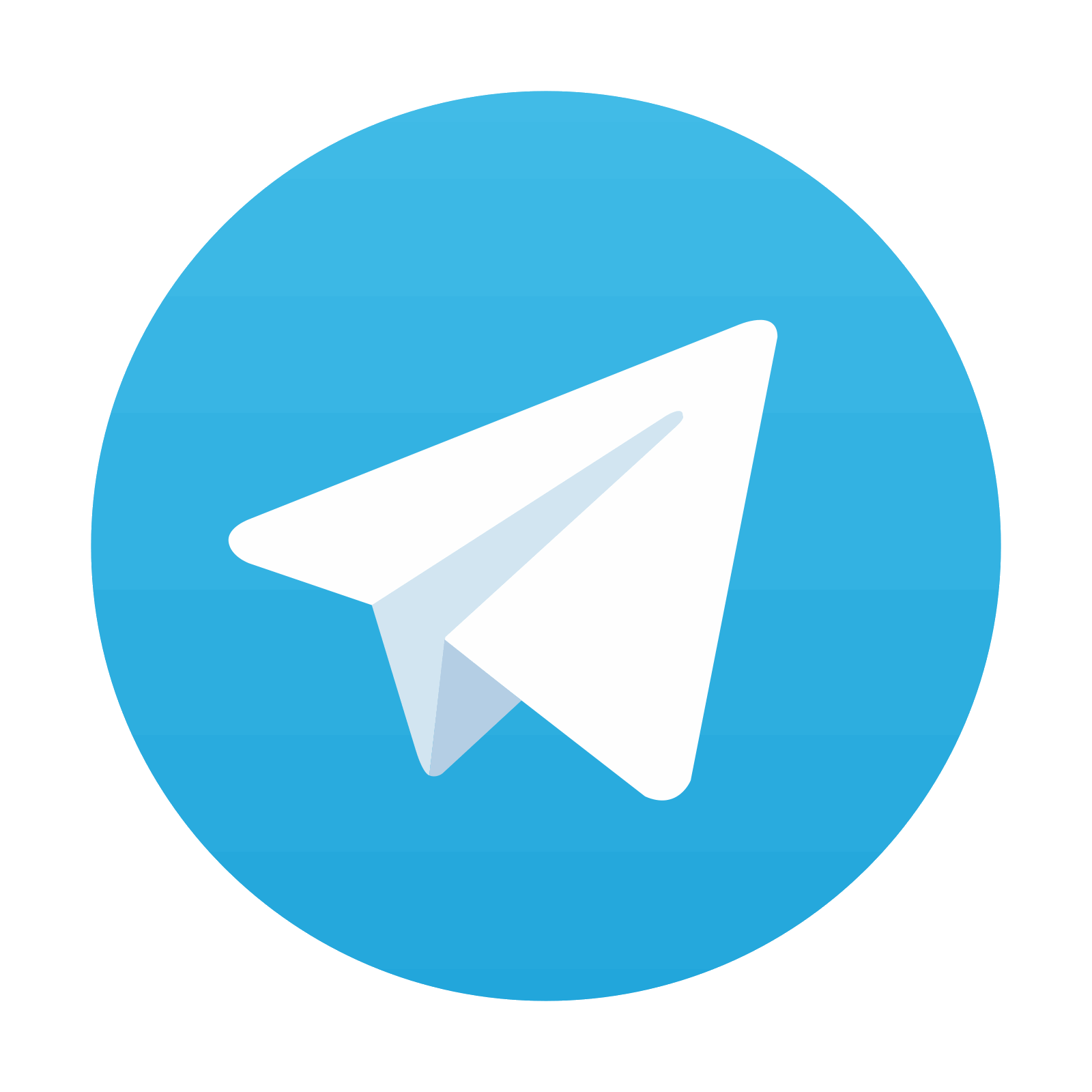
Stay updated, free articles. Join our Telegram channel
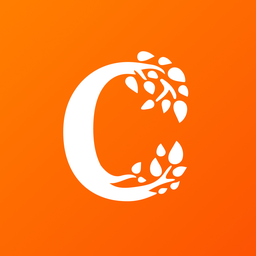
Full access? Get Clinical Tree
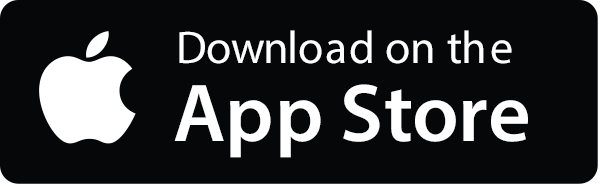
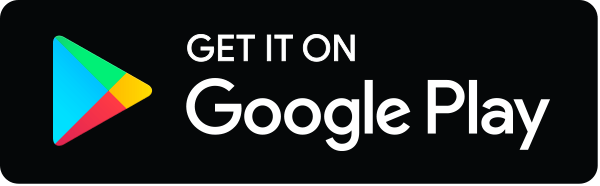