Physical Basis of Modern Radiotherapy: Dose and Volume
Paul Keall
José Belderbos
Feng-Ming (Spring) Kong
About 60% of cancer patients receive radiation therapy (RT),1 making radiation the most common “drug” (or more appropriately termed, prodrug) in cancer treatment. Radiation affects cell function by either directly ionizing intracellular targets or creating free radicals near the intracellular targets, which can then react with and disrupt normal cell processes. The most important and understood intracellular target is deoxyribonucleic acid (DNA), where single-and double-strand breaks can occur resulting in cell damage and cell death.
Radiotherapy is an example of individualized therapy. The delivery of the cytotoxic agent—radiation—varies from patient to patient as a result of the changing tumor and normal anatomy. Determining the appropriate dose and tumor volume to treat, choosing the appropriate beam shapes and energy for the treatment plan, and executing the treatment plan during radiation delivery involves a dedicated and coordinated effort by the treatment team, including the radiation oncologist, medical physicist, dosimetrist, and radiation therapist. Lung cancer is a particular challenge for radiotherapy given the number of radiation-sensitive tissues that ideally should be avoided—the lungs, esophagus, heart, spinal cord, mainstem bronchii, pulmonary vessels, brachial plexus and ribs, as well as the large changes in tissue density that challenge accurate calculation of dose to these structures.
This chapter describes the physical basis of dose and volume in radiotherapy and is divided into four main parts: imaging for volume delineation in RT, treatment planning, dose and fractionation, and treatment delivery. Although imaging, planning, and delivery have previously been three distinct processes in radiotherapy, with the emergence of image-guided therapy and adaptive therapy, the imaging-planning-delivery processes are merging.
IMAGING FOR VOLUME DELINEATION IN RADIATION THERAPY
Preparation In radiotherapy for lung cancer, it is important to keep geometrical uncertainties such as patient tumor delineation, organ motion, and setup variation to a minimum. The requirements on accuracy, dependent on the dose prescribed, are dictated by the purpose of the irradiation. In case a palliative treatment for lung cancer is prescribed, the requirements of the radiotherapy treatment preparation (traditional two dimensional [2D] using x-ray simulator) and delivery are relatively uncomplicated compared with three-or four-dimensional radiotherapy techniques used in radical or curative settings. Three-dimensional (3D) conformal radiotherapy is based on computed tomography (CT) scan treatment preparation and individualized treatment fields. Four-dimensional (4D) CT scans provide information on respiratory-induced tumor motion, which can be used for patient-specific treatment preparation.
Immobilization To reassure stable and reproducible patient positioning during treatment preparation and execution, the patient is placed (as comfortably as possible) on a flat couch with both hands stable above the head using immobilization devices (e.g., T-bar device, forearm support, alpha cradle, Wing Board, or vacuum mattress). These immobilization devices, sometimes personalized, will decrease the setup errors and reduce the daily setup uncertainty.2 Available data support the use of an immobilization device to achieve stable and reproducible patient positioning during treatment planning and delivery.3,4,5The delivery of hypofractionated treatment fractions (stereotactic body irradiation; see Chapter 43) or gated treatments (irradiation to a restricted portion of the respiratory cycle) takes more beam-on and total machine time. For these treatments with prolonged treatment time, additional immobilization devices such as a stereotactic body frame and/or abdominal compression might be used to increase intrafraction stability or decrease respiratory tumor motion. Other devices such as head and knee cushions are frequently used to ensure maximum comfort and thus improve the position reproducibility. However, results of studies reveal that the setup accuracy (with respect to the bony anatomy) using a stereotactic body frame is of the same order (2 to 4 mm [1 standard deviation]) as without using such immobilization devices.6,7
The training and attention to detail of the treatment team is important in maintaining anatomic reproducibility throughout the treatment course.
Simulation For radiotherapy treatment preparation of a palliative schedule, an x-ray simulation session using a diagnostic x-ray generator (that has the same geometrical features of the linear accelerator) can be sufficient, although a growing number of centers are performing CT simulation for all patients, including those treated for palliation. During simulation the patient is in treatment position, and the radiation oncologist will determine the treatment field borders. Tumor motion information gathered during fluoroscopy in the cranial-caudal direction may be used. The anterior and lateral laser lines and/or the treatment field borders will be marked on the patient (sometimes using tattoos) to be used during daily patient setup.
For 3D conformal radiotherapy, a CT scan of the thorax in treatment position using a CT simulator is necessary. A CT simulator is a CT scanner with a laser system to align the patient and specific software. Laser lines will be marked on the patient, and the isocenter of the laser lines will be made visible on the planning CT scan. This so-called planning (or simulation) CT scan provides information on the 3D tumor extensions (and the normal tissues surrounding the tumor) and will be used to determine the optimal beam arrangements and calculate the dose distribution. An axial planning CT slice is shown in Figure 40.1. This planning CT is normally performed from middle/upper neck (the level of C2-C3) to middle abdomen (L2-L3), so that the whole lung is included for dosimetric estimation. Intravenous contrast is recommended for patients with tumors near the mediastinum and/or pathological lymph nodes. This is helpful for differentiating centrally located tumors and enlarged lymph nodes in the hilum and mediastinum from adjacent vessels.8,9 The use of intravenous contrast may not be that critical if a recent contrast-enhanced diagnostic CT scan or positron emission tomography (PET)-CT simulation is available,10,11 or for a peripherally located tumor without positive lymph nodes. CT scans with a slice thickness of 2 to 3 mm are recommended to enable accurate gross tumor volume (GTV) delineation, better recognition of nodal structures, and high-resolution digitally reconstructed radiographs (DRR) to be generated, which in turn may allow for the omission of a separate simulation step.12 Study of a small patient series indicated that the impact of contrast on dose estimation is less than 2% to 3% of the prescription dose.13
![]() FIGURE 40.1 Views from a planning CT scan. Note the flat tabletop used for radiation therapy imaging, the absence of the patient’s arms as they are above their head to avoid being radiated during treatment, and the foam mold surrounding the patient to assist with immobilization. The gross tumor volume (red) and planning target volume (pink) are shown. (See color plate.) |
Multiple laser setup points are placed on the patient to ensure that the patient is approximately in the same position each day for treatment. The distributions of treatment setup errors measured against DRRs obtained in the CT simulation are similar to previously obtained distributions measured against simulator films, and DRRs are well suited for setup verification. A verification simulation under conventional fluoroscopic simulator may be omitted.12 However, the tumor motion should be checked for estimation of planning target volume (PTV) margin and adequate target coverage if the simulation and treatment were performed under free breathing (see section on “Motion Considerations” later in this chapter).
To minimize simulator setup errors, a treatment isocenter (preferably located within the treatment target) should be defined at the time of treatment planning. Volumetric information from spiral CT scans is superior for defining target volumes rather than data derived using axial CT scans.14 However, it should be recognized that movement-induced artifacts for mobile organs or targets can arise during most CT scan techniques.15 The possibility of time-related displacements and treatment-related changes of target volumes that had been defined prior to a 4- to 7-week course of radiotherapy has not been adequately studied,16 and is an import topic of future research.
Determination of Treatment Volumes The modern 3D conformal radiation technique (CRT) requires precise definition of the treatment target and organs at risk. This is different from the traditional 2D practice. Definitions: the GTV includes the primary tumor (GTV-P) and the nodal GTV (GTV-N). The clinical target volume (CTV) accounts for microscopic (subclinical) disease. The PTV incorporates tumor delineation uncertainty, organ motion, setup variation, and intrafraction stability.
Lymphatic spread is an important pathway of progression of non-small cell lung cancer (NSCLC), along with local spread and distant metastasis. The probability of lymph node invasion is dependent on the site of the primary lung tumor, tumor stage, and histology. Before radiotherapy treatment starts, the extent of lymph node spread needs to be determined as correctly as possible. In the past, it was impossible to stage lymphatic spread accurately, and large radiation fields were used to encompass lymph node areas that were clinically and radiologically uninvolved. These large fields, called elective nodal irradiation (ENI), were covering, for example, the homolateral supraclavicular fossa, the hilar region, or the mediastinal lymph nodes. The use of these large irradiation fields increased the volume of normal tissues irradiated considerably, which led to more toxicity and prevented successful dose escalation. The influence of these large elective fields on treatment outcome was never established in randomized trials. Obviously, the extent and quality of the staging examinations performed pretreatment influences the need for ENI.
Delineation of Gross Tumor Volume Target delineation of GTV is essential for 3D CRT. The target volume definition should follow the guidelines of the International Commission of Radiation Units and Measurements (ICRU). The GTV includes the GTV-P, and the GTV-N; GTV-N includes any abnormally enlarged regional lymph node (hilar and/or mediastinal), as well as mediastinal and/or supraclavicular nodes enlarged 1 cm or more in the short axis,12 unless metastases have been excluded by other means such as mediastinoscopy or18 F-fluorodeoxy-glucose (FDG)-PET scanning. GTV-N should also include lymph nodes that are not enlarged but have been documented by mediastinoscopy/endoscopic ultrasound (EUS)/endobronchial ultrasound (EBUS) as being pathologically involved or have higher FDG activity than the mediastinal blood pool. The measured diameter of tumors in lung parenchyma or mediastinum is highly dependent on the window width and level chosen during the delineating process.17 Lung primary tumors should be outlined under the standard lung window, whereas the nodal disease and centrally located tumors are better contoured under mediastinum window/levels. The specific window widths and levels may vary with the CT scanner, software used, and the technique, with lung settings at W = 400 to 1600, L = −400 to =600, and mediastinum settings ranges W = 400 to 1000, L = 0 to 50.17,18,19 One study found that the best concordance between measured and actual diameters and volumes was obtained with the following settings: W = 1600 and L = −600 for parenchyma, and W = 400 and L = 20 for mediastinum.19 It is preferable that such parameters be specified in each center with the help of a radiologist, and be preset in treatment planning workstations, to improve consistency in contouring.
Depending on the tumor location, the adequate window and level setting should be viewed to delineate the GTV. For example, when the tumor is surrounded by lung tissue only, the lung level and window CT settings should be used. For lymph nodes and lung tumors invading the mediastinum or chest wall, only the window with mediastinum level and window settings should be viewed. The use of 4D CT scanning may reduce delineation uncertainties because of an improved visualization of the tumor shape (see section on “Motion Considerations”).
Determination of Clinical Target Volume The CTV accounts for microscopic (subclinical) disease. This subclinical area may involve a region around the GTV as well as regional lymph node areas that were not documented to have adenopathy or proved by mediastinoscopy to be involved. It is controversial what this volume should include. It is extremely challenging to estimate the extent of CTV. Generally, the size of margin added to the GTV to account for microscopic extent has been arbitrary, ranging from 5 to 8 mm. Giraud et al.19 examined NSCLC surgical specimens with adenocarcinoma (ADC) and squamous cell carcinoma (SCC) histology. The mean value of microscopic extension was 2.7 mm for ADC and 1.5 mm for SCC. A 5-mm margin covers 80% of the microscopic disease extension for ADC and 91% for SCC. To have 95% confidence that all tumor is included in the clinical target volume, a margin of 8 and 6 mm must be chosen for ADC and SCC, respectively. Grill et al.20 analyzed microscopic disease extension in 35 cases and reported that in a GTV contoured using CT lung windows, the margin required to cover microscopic extension for 90% of the cases was 9 mm (9, 7, and 4 mm for grade 1 to 3, respectively).
The concept of nodal CTV includes CTV around the GTV-N and the microscopic disease within clinically normal nodes. Yuan et al.21 studied extracapular extension (ECE) in 243 cases and concluded that ECE extent was related to lymph node size, stage, and differentiation. The extent of ECE was 3 mm in 95% of the nodes. It may be reasonable to recommend 3-mm CTV margins for pathologic lymph nodes (GTV-N) <20 mm and more generous margins for lymph nodes ≥20 mm. The
microscopic disease extension to the clinically uninvolved nodal regions has been a topic of debate and has been evolving over the last several years. During the 1980s, this volume routinely included mediastinum, bilateral hilum, and the supraclavicular areas. Emami et al.22 reported that inclusion of the contralateral hilum, the mediastinum, and supraclavicular lymph nodes in the field did not affect local control or survival. In the 1990s, most had eliminated the contralateral hilum and/or the supraclavicular areas. However, whether or not the ipsilateral hilum lymph node group was included per protocol did appear to affect local control and survival.23 Recent results with 3D CRT without intentionally including these elective nodal regions showed a low incidence (0% to 7%) of isolated nodal failure in several dose escalation studies.16,24,25,26
microscopic disease extension to the clinically uninvolved nodal regions has been a topic of debate and has been evolving over the last several years. During the 1980s, this volume routinely included mediastinum, bilateral hilum, and the supraclavicular areas. Emami et al.22 reported that inclusion of the contralateral hilum, the mediastinum, and supraclavicular lymph nodes in the field did not affect local control or survival. In the 1990s, most had eliminated the contralateral hilum and/or the supraclavicular areas. However, whether or not the ipsilateral hilum lymph node group was included per protocol did appear to affect local control and survival.23 Recent results with 3D CRT without intentionally including these elective nodal regions showed a low incidence (0% to 7%) of isolated nodal failure in several dose escalation studies.16,24,25,26
There is lack of solid evidence currently existing for either pro or con ENI.27 Although most of the published results from the cooperative groups used the elective radiation to most of the mediastinum regions, the newly activated protocols for NSCLC have a tendency to omit such radiation (Radiation Therapy Oncology Group [RTOG] 0617 and European Organisation for Research and Treatment of Cancer [EORTC] trials). If the treating physician believes an elective nodal area (primarily of the mediastinum or ipsilateral hilum) is indicated, it should be contoured and identified on CT scans. The atlas of nodal regions from University of Michigan is an important tool to determine the anatomic area of the lymph nodes involved.28
Determination of Planning Target Volume A range of geometrical uncertainties are associated with radiotherapy of lung cancer patients, such as target definition uncertainties, setup errors, organ motion, and anatomical changes. Errors (uncertainties) made during treatment preparation (e.g., target definition errors, or a nonrepresentative planning CT scan) will be made only once. In the absence of correction protocols, these errors will then be present during every treatment fraction. Errors made during treatment delivery of fractionated radiotherapy (e.g., misalignment of the patient), on the other hand, will be made several times and are likely to be different every day. Errors that are identical for every fraction are called systematic errors, whereas errors that vary day-by-day are called random errors. The dosimetric effect of systematic and random errors is different; random errors blur the cumulative dose distribution, whereas systematic errors shift the cumulative dose distribution.
To account for geometrical uncertainties, safety margins are applied around the CTV, thereby defining the PTV and thus irradiating a larger volume (Fig. 40.2). Often, separate margins are used for patient positioning uncertainty and for organ motion, called the setup margin (SM) and the internal margin (IM), respectively. As external error sources and internal error sources are generally not correlated, a linear addition of these margins, however, is not correct. Alternatively, the CTV is directly expanded to the PTV. Several margin recipes have been proposed in the literature with different objectives. For example, van Herk et al.29 showed that the margin around the CTV for conventional fractionation should be 2.5 times the standard deviation of all the systematic error plus 0.7 times the standard deviation of all the random error to make sure that 90% of the patient’s GTV will receive at least 95% of the dose. Note that the distribution of systematic and random uncertainties is institution dependent and, thus, need to be carefully evaluated for each department.
Traditionally, a uniform 7- to 10-mm margin was used to extend the CTV in all directions to form the PTV. However, because the respiratory induced tumor motion is nonuniform and patient dependent, the PTV should also be nonuniform and patient dependent. Moreover, detailed analysis of several sources of geometrical uncertainties30,31,32,33 has revealed that such margins are too small in the absence of image guidance and associated correction strategies.
The use of 4D CT scanning technique allows for accurate estimation of tumor motion, although over a short time interval, and generates individualized margins. Various approaches for the incorporation of 4D CT scanning in treatment planning have been published recently.34,35,36,37 In general, the use of 4D scanning in combination with gating, or the reconstruction of a time-averaged tumor position (midventilation CT scan), allows an important reduction of the PTV (Fig. 40.2) compared with the PTV for conventional free breathing CT scanning or the internal target volume (ITV). The ITV concept (note that the ITV is defined differently from the original ICRU definition) covers the entire tumor motion, in one breathing cycle, and therefore, may overestimate the influence of the motion on the tumor dose.38 Additionally, critical normal tissues where knowledge of the radiation dose is important and/or dose should be minimized need to be carefully and consistently defined. An example of normal structure definition is shown in Figure 40.3.
The Role of the Positron Emission Tomography Scan on Target Delineation FDG-PET has had a significant impact on the delineation of the GTV for NSCLC, because it images metabolically active tumor cells, and identifies pathological lymph nodes more accurately than CT scanning.39,40,54 A metaanalysis of 39 FDG-PET studies examined
the diagnostic accuracy of FDG-PET scanning in patients with NSCLC using stratification for mediastinal lymph node size on CT scan.41 Based on this analysis, the sensitivity of the FDG-PET scan for enlarged mediastinal lymph nodes was 91% and 75% for not-enlarged lymph nodes. FDG-PET also improved the staging of solitary pulmonary lesions42 and extrathoracic spread of the disease. Another advantage of FDG-PET scanning is the possibility to distinguish tumor from collapsed lung or mediastinal structures. In addition, evidence is accumulating that the standardized uptake value (SUV) of FDG-PET tracer in the primary tumor is an important prognostic factor for radiotherapy treatment outcome.43,44,45,46
the diagnostic accuracy of FDG-PET scanning in patients with NSCLC using stratification for mediastinal lymph node size on CT scan.41 Based on this analysis, the sensitivity of the FDG-PET scan for enlarged mediastinal lymph nodes was 91% and 75% for not-enlarged lymph nodes. FDG-PET also improved the staging of solitary pulmonary lesions42 and extrathoracic spread of the disease. Another advantage of FDG-PET scanning is the possibility to distinguish tumor from collapsed lung or mediastinal structures. In addition, evidence is accumulating that the standardized uptake value (SUV) of FDG-PET tracer in the primary tumor is an important prognostic factor for radiotherapy treatment outcome.43,44,45,46
![]() FIGURE 40.3 Dose-limiting normal tissues to avoid and/or limit radiation dose. Target volume delineation is performed on the entire 3D (or 4D) data set. Right lung: blue; left lung: green; heart: pink; esophagus: green; spinal cord: brown; main airways: yellow. The target volumes can be seen in Figure 40.1. (See color plate.) |
The introduction of FDG-PET has a large influence on selecting patients for radical radiotherapy treatment. Several studies have demonstrated that FDG-PET scan implementation in radiotherapy treatment planning influenced the delineation of the GTV.47,48,49,50,51,52,53,54,55,56,57,58,59,60,61 The most common findings of the studies have been changes of GTV and PTV based on the PET image, which subsequently might improve the estimated tumor control probability and normal-tissue complication probability.55,56 PET imaging can provide a more accurate representation of the 3D volume-encompassing motion of model tumors and has the potential to provide patient-specific motion volumes for an individualized ITV.57 For patients, the PET-delineated target volume seemed to be consistent with the ITV determined from all sets of gated-CT and regular spiral CT images. PET use in estimating the individual ITV is further addressed by Fernando et al.58 Additionally, implementing matched CT-FDG-PET reduced interobserver and intraobserver variation in target delineation significantly with respect to CT only.52,59
Steenbakkers et al.33 observed a reduction in the interobserver variability of more than 50% in the standard deviation of the GTV delineations if FDG-PET information coregistered with CT was used. Preferably, the tumor delineation should be based on a matched CT-FDG-PET scan (with both examinations in treatment position) and performed by a radiation oncologist experienced in lung cancer treatment in the presence of a detailed delineation protocol and adequate delineation software.33
It is clear that PET has significantly changed the treatment planning of NSCLC patients, and is considered to be a state-of-the-art technique. Images from a planning FDG-PETCT scan are shown in Figure 40.4.
Issues remain, however, with the use of PET in target delineation, some of which were discussed in a recent journal editorial.60 For example, the determination of the edges of the tumor in the metabolically active area on PET is not straightforward. Most of the studies that were mentioned used an arbitrary threshold value of the maximum intensity (30% to 50%) in the PET-avid area or a standardized uptake value (range: 2 to 5). This threshold, however, was generated from phantom studies of limited target size,61 and is not correct for most patients with NSCLC.62 Several phantom studies have attempted to determine optimal threshold values, with differing results. Researchers from Beaumont Hospital63 performed a series of sphere phantom studies to determine an accurate and uniformly applicable method for defining a GTV with
FDG-PET. They found a strong linear relationship between the threshold SUV and the mean target SUV. Recent studies from patients further confirmed that the threshold for the best match of CT-GTV averaged 20% to 25% (range: 10% to 50%) ITV 15% to 20% (range: 10% to 50%), depending on the tumor size, activity, and location.58
FDG-PET. They found a strong linear relationship between the threshold SUV and the mean target SUV. Recent studies from patients further confirmed that the threshold for the best match of CT-GTV averaged 20% to 25% (range: 10% to 50%) ITV 15% to 20% (range: 10% to 50%), depending on the tumor size, activity, and location.58
![]() FIGURE 40.4 PET images overlaid on the CT scan from a treatment planning PET-CT simulation session. For radiation oncology, a critical step is the delineation of the tumor boundaries that are necessary for determining the shape of the radiation treatment beams. (See color plate.) |
Unfortunately, FDG-PET has its limitations. Firstly, the spatial resolution of FDG-PET images is poor (4 to 6 mm) compared with CT scan images.57,64 Therefore, the increased FDG uptake should be used for localization of the tumor, not for defining boundaries, except for the tumor-atelectasis region. In a study by Steenbakkers et al.33 10% of the lymph nodes with increased FDG uptake were missed by the radiation oncologists. One explanation might be that the FDG-PET report was not read accurately enough by the radiation oncologists. Furthermore, for some lymph nodes, the level and window settings of the FDG-PET had to be changed before they were visible.
Another challenging issue of using PET for treatment planning is to register the PET image accurately when a PET-CT simulator or hybrid PET-CT is not available. An ideal registration method, which uses the entire volume of image data (i.e., intensities of the image voxels) for matching of “mutual information” and displays the two modalities simultaneously, would significantly improve the accuracy of PET in target delineation.
Motion Considerations Tumor motion caused by breathing and heartbeat is a topic of many investigations. Tumor motion could be rather substantial and heterogeneous, ranging from a few millimeters to 25 mm based on tumor location, size, and patient condition.65,66,67,68,69 The greatest tumor motion is seen in the cranial-caudal direction. Information on the individual patient’s lung tumor motion is necessary to determine individual margins.66 It is critical to accurately estimate the motion for each tumor, so that the treatment planning is optimized for target coverage and normal-tissue sparing. With the advancement of imaging techniques, several strategies are now available to approach this goal. These include, but are not limited to, visualization under fluoroscopy, respiratory-gated CT simulation, and 4D CT simulation.
Tumor motion can be visualized under a conventional simulator with fluoroscopy, and the extent of motion can be estimated for each tumor.70,71 With quiet respiration, the maximum tumor movement in the craniocaudal direction was 12 mm, and the mediolateral and dorsoventral direction was 5 mm in a study by Ekberg et al.70 Other reported movements ranged from 0 to 12.8 mm in the superior-inferior direction, 0 to 3.2 mm in the lateral direction, and 0 to 4.4 mm in the anterior-posterior direction.71 Large variation in GTV motion from patient to patient was observed, especially in the superior-inferior direction, for which interpatient variability was >10 mm.
Tumor motion seemed to be unique for each patient and in each dimension. A standard uniform PTV margin of 10 or 15 mm is inappropriate. Motion check under fluoroscopy can provide an estimation of the internal motion in each patient. This method is simple; however, it is limited by the facts that (a) some tumors can be difficult to visualize with fluoroscopy x-rays; (b) diaphragm motion may not correlate with tumor movement; and (c) the shape change of the tumor cannot be measured clearly. Additionally, fluoroscopy generally overestimates the mobility of visible lung tumors and may result in irradiation of unnecessarily large target volumes.72 Even if an individualized margin is obtained from the fluoroscopy, an
ideal PTV cannot be guaranteed from GTV because the free-breathing CT images can be taken in any phase of the breathing cycle when the target volume is scanned. For example, assume the target is scanned at the end of exhale (the target in the most superior position), a uniform expansion would include unnecessary normal tissue superiorly and would not cover the inferior part of the target adequately.
ideal PTV cannot be guaranteed from GTV because the free-breathing CT images can be taken in any phase of the breathing cycle when the target volume is scanned. For example, assume the target is scanned at the end of exhale (the target in the most superior position), a uniform expansion would include unnecessary normal tissue superiorly and would not cover the inferior part of the target adequately.
With the wide availability of CT simulation, techniques with modifications to the CT simulation procedure have been proposed to better define the treatment target. The most common of these is respiratory correlated 4D CT.73,74,75,76 A multislice CT scanner is typically used for 4D CT. In the cine mode, the CT scanner scans the same table position for one or more whole respiratory cycles, and then moves to another table position. Computer programs then sort out the images according to the respiratory phases and reconstruct the 3D CT images at each phase. Usually, 10 sets of images of 10 different phases are obtained for one simulation.
An ITV can then be generated by combining the GTVs of different phases with a CTV margin. Theoretically, using 4D CT images should be the best way to approach ITV accurately. The challenge of this technique is the burden for the treatment planning computer to handle the large amount of data and for the radiation oncologists to delineate/check the target in each image set. Also, there are several artifacts found using current generation 4D CT scan technology77,78,79 that could be improved through breathing training, improved acquisition techniques, and/or better post-acquisition reconstruction methods.
Another technique referred to as the mid-ventilation (MidV) CT scan generates a single 3D CT frame from 4D CT images representing the tumor closest to its mean tumor motion position.37 Using this MidVCT scan, the systematic contribution caused by breathing motion can be reduced to nearly zero, permitting a reduction of the treatment margin. The expected dose-blurring effect of the respiration can be accounted for in the CTV to PTV margin. Because of the presence of the wide beam penumbra in lung, Engelsman et al.80 and Witte et al.81 reported that if a treatment plan is designed for the tumor in its (time-weighted) average position during the respiration cycle, a good dose coverage is still obtained even if the tumor is not fully within the PTV during a small part of the breathing cycle.
One or more phases of the 4D CT scan (e.g., the end exhale, or scans near and including end exhale) can be used for respiratory-gated treatment planning/delivery. Alternatively, a gated CT scan can be acquired by triggering the CT scanner to acquire imaging data when the patient’s respiratory signal crosses a predetermined threshold (e.g., near end exhale).
To reduce tumor motion, several strategies have been developed and tested. These fall within two classes: abdominal compression and breath-hold techniques. Abdominal compression was originally developed for stereotactic irradiation of small lung and liver lesions by Lax and Blomgren82,83,84 and has been used elsewhere.6,85,86,87,88,89,90,91 The technique employs a frame with an attached plate that is pressed against the abdomen. The applied pressure to the abdomen reduces diaphragmatic excursions, while still permitting limited normal respiration. The accuracy and reproducibility of both the body frame and the pressure plate have been evaluated by several groups, with a comprehensive assessment reported by Negoro et al.86 Abdominal compression has predominantly been applied to early stage lung and liver tumors without mediastinal involvement or nodal disease, although the technology is also applicable to conventional lung treatments.
The most widely used breath-hold methods are active breathing control (ABC) described by Wong et al.92,93 and deep inspiration breath hold.94,95,96 Using breath-hold devices, CT simulation and treatment delivery can be performed at the same fixed level of controlled breathing; thus, the target is considerably reduced during treatment planning and delivery. In addition to motion reduction, breath-hold techniques have the advantage that the lung volume is expanded by 30% to 50%, thus for a given beam aperture, the fraction of lung being irradiated is smaller. However, implementation of breath-hold techniques requires patient selection for compliance and increases the time of the imaging and treatment procedures.
Other motion management techniques, such as using gated treatment at a specifically defined phase window when the patient breathes freely, or tumor tracking (in which the radiation beam follows a measurement or estimate of the tumor motion) are clinically available and, with improved integration of image guidance with treatment delivery, are likely to become more widespread.
TREATMENT PLANNING
Aiming to maximize the radiation dose to the target volume while minimizing dose to surrounding normal tissues, the 3D CRT is now the standard technique of RT in lung cancer. Planning and delivery of RT is a multistep process that is individualized for each patient. Literature-based EORTC guidelines on each of these steps for 3D CRT in the setting of clinical practice and clinical trial have been published.97 The process of RT planning includes dose/fractionation, immobilization/localization, target and normal-tissue delineation (including tumor and important normal structures), radiation beam design, and treatment delivery.
Beam Arrangements/Aids and 3D CRT The design of the radiation beam field or aperture depends on the treatment volume, with or without inclusion of ENI. With ENI, the beam selections are limited by the extensive volume of the target—often large fields aimed from the anterior and posterior (AP/PA) direction of the patient in parallel-opposed fashion. The AP/PA beam arrangement is limited by the tolerance dose of the cord, thus, one or more “off-cord” fields are always needed for radical treatment when a dose of 60 Gy or higher is needed. Although the nodal volume was traditionally determined by anatomical landmarks located on simulator radiographs or reconstructed coronal CT planes (as it was for 2D planning), the nodal regions should be contoured consistently,
so the PTV can be defined and the plan of 3D CRT can be generated. This technique offers the potential advantage of improved dose delivery to a target volume (as opposed to a point dose calculation in 2D planning, historically).
so the PTV can be defined and the plan of 3D CRT can be generated. This technique offers the potential advantage of improved dose delivery to a target volume (as opposed to a point dose calculation in 2D planning, historically).
Using 3D CRT with selective ENI or without ENI, the PTV is to be treated with any combination of coplanar or noncoplanar 3D conformal fields shaped to deliver the specified dose while restricting the dose to the normal tissues. Field arrangements are determined by 3D planning to produce the optimal conformal plan in accordance with volume definitions. Multiple coplanar or noncoplanar field arrangements are preferred, but AP/PA fields are still used, if appropriate, on an individual basis. The beam directions are selected by the use of the beam’s eye view (BEV) tool. Target and normal structures are viewed from different directions in planes perpendicular to the beam’s central axis using BEV. The beam shape is then modified by designing a block or a block substitute called a multileaf collimator (MLC) shaper, which will allow full dose to the PTV but minimize the dose to surrounding normal tissue. To cover the entire PTV with the prescription dose, an additional margin (block margin) should be added to each aperture. The block margin is to compensate the penumbra at each beam edge; and often 5 to 10 mm is needed for an adequate coverage at the peripheral part of PTV. It should be kept in mind that this margin width is dependent on the number and arrangement of fields and the specific technique used. For coplanar arrangement of multiple beams, smaller lateral margins are needed for adequate PTV coverage (i.e., relatively larger superior-inferior margins for each beam).
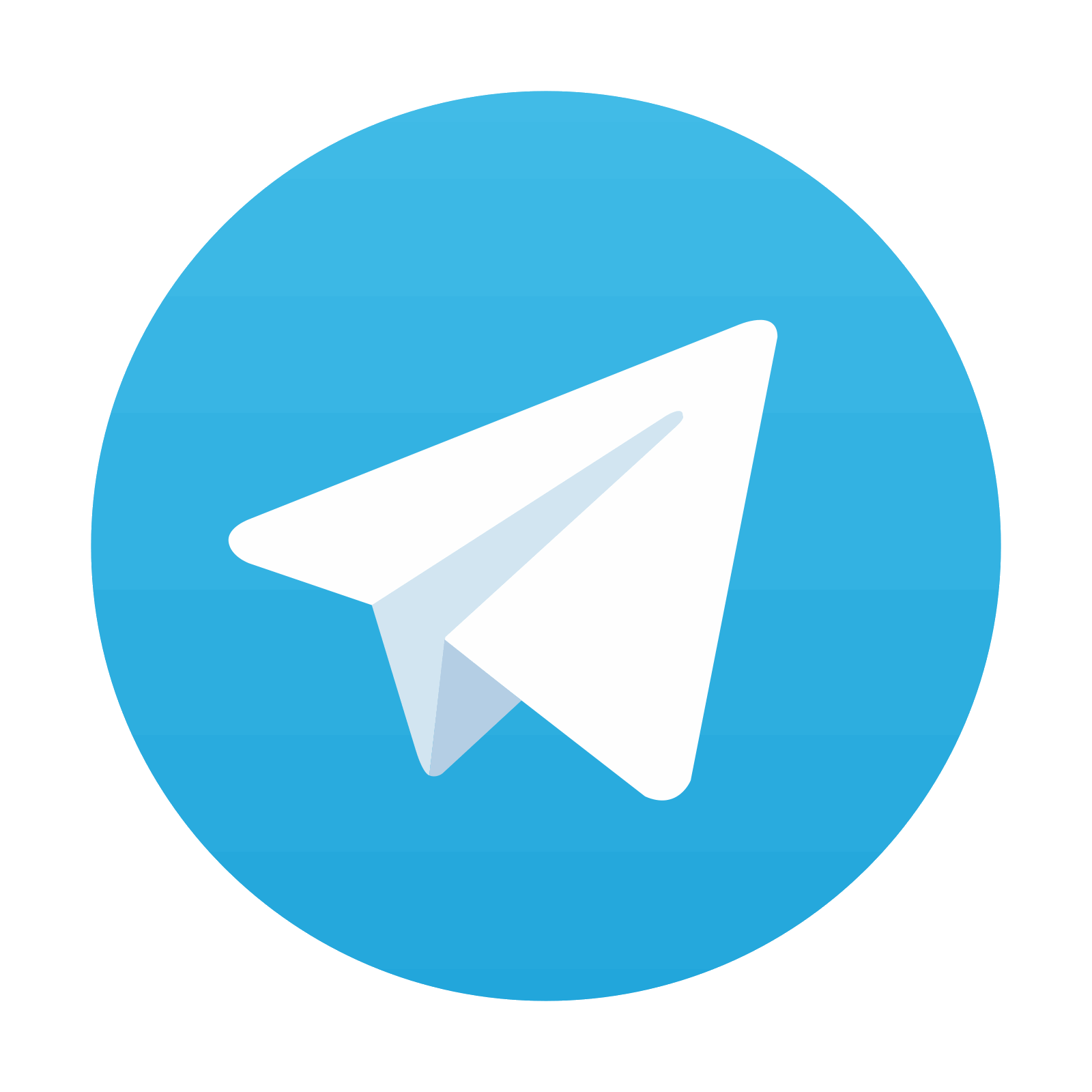
Stay updated, free articles. Join our Telegram channel
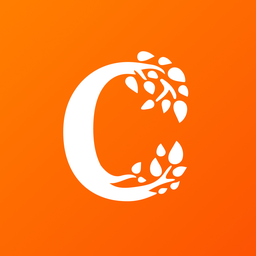
Full access? Get Clinical Tree
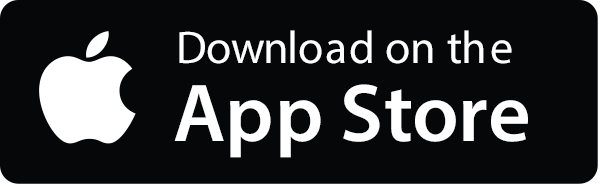
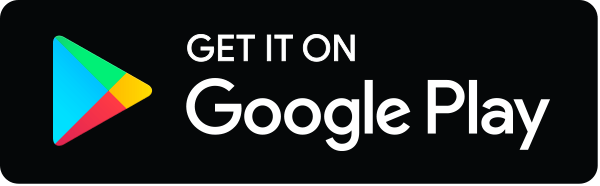