Fig. 3.1
The apex of the heart when viewed from above in systole and diastole; note the position of the valves and their relationships (Modified from Dabbagh (2014). Published with kind permission from © Springer, 2014. All Rights Reserved)
Let’s discuss here the three main myocardial jobs in more detail (i.e., electrical function, ECC, and contractile function).
Electrical Function of the Myocardium: Action Potential
The electrical part of cardiac function is done through a well-organized and highly specialized electrical network; this network has two main cell types (Fig. 3.2):


Fig. 3.2
Cardiac conductive system and its elements; in left, see the relationship of normal electrocardiography with the elements of the system (Modified from Dabbagh (2014). Published with kind permission from © Springer, 2014. All Rights Reserved)
“Impulse-generating cells” which have excitatory function and produce impulse; these cells mainly compose the sinoatrial (SA) node.
The SA node lies in the cephalic portion of the posterior wall of the right atrium just near to the orifice of SVC. The SA node has a complex architecture leading to heterogeneous electrical activity throughout the SA node. Also, pacemaking cells, especially in the SA node, have a number of specific specifications:
Higher rate of spontaneous beating
Faster activation of the funny current (I f) which is described later
Greater density of the funny current (Mikawa and Hurtado 2007)
“Conductive cells” which are a well-defined network for conduction of the impulse and is composed of:
The atrioventricular (AV) conduction pathways.
The atrioventricular (AV) node which lies just superior to the endocardial cushions, in the inferior part of the interatrial septum and anterior to the coronary sinus foramen.
The His bundle.
The right and left branches of His bundle.
The network of Purkinje fiber cells also known as Purkinje fiber network; the Purkinje fiber network is an extension of the His bundle distributed all over the ventricular tissue, in such a way to propagate and to conduct the electrical impulse throughout the ventricles as fast as possible (Desplantez et al. 2007; Dun and Boyden 2008; Atkinson et al. 2011).
Action Potential in Cardiac Cells
Certain ions, ion currents over both sides of the myocardial cell membrane and the cell membrane itself (including its integral structures like membrane channels, receptors, and enzymes), and, also, a number of internal cell structures work together to produce the electrical activity of myocardial cells. Electrical activity achieved as a result of different ion currents (Na+, K+, and Ca2+) and activation of certain receptors or enzymes can alter this activity. In fact, the ion currents have the main role, and other factors like sympathetic and parasympathetic effects do not exert roles in generation of action potential; if we denervate the heart of an animal in lab, action potential and impulse generation would not be stopped but can affect the generation and shape of the action potentials.
Generation of action potential is essential for producing mechanical activity in the muscular wall of atria and ventricles, depolarization for contraction and repolarization for relaxation. For this, the action of the heart is called electromechanical activity.
Generally, there are two types of action potential in the heart: fast response action potential in the myocardium and Purkinje system and slow response action potential in nodal cells. Myocardial action potential consists of five sequential phases: phase 0 to phase 4. These phases compose together the electrical wave of myocardial cells known as action potential, “AP”, which are described here in Table 3.1 and Fig. 3.3.

Table 3.1
Action potential in myocardial cells

Cardiac Automaticity and Its Mechanism(s)
How does the pacemaker activity in the cardiac cells happens? For answering this question, we should look for the main difference between “myocardial cells” and “pacemaker cells” regarding action potential.
Action potential (AP) in cardiac pacemaker cells
Although the main mechanisms in AP of cardiac pacemaker cells are similar to those in myocardial cells, there are a number of differences:
- 1.
Resting membrane potential in pacemaker cells is higher than myocardial cells; i.e., while resting potential is about −90 to −80 in myocardial cell population, it is about −60 to −50 in cardiac pacemaker cells; one of the main mechanisms for this upper level of resting potential in pacemaker cells is Na+ influx during repolarization known as the funny current abbreviated as I(f) (DiFrancesco and Noble 2012; Papaioannou et al. 2013; Weisbrod et al. 2013).
- 2.
There are two main Ca2+ channels in the heart tissue: T (transient) type Ca2+ channels (abbreviated as I CaT) and L (long-lasting) type Ca2+ channels (abbreviated as I CaL); both I CaT and I CaL are categorized under voltage-gated Ca2+ channels (VGCC) and have their role in impulse generation (i.e., SA node) and atrioventricular impulse conduction (i.e., AV node); though I CaT typically diminishes in the normal adult heart, they have an active role in the final segments of repolarization and play their role in combination with I(f) to produce prepotential steep which is in fact the “pacemaker potential”; I CaT has two isoforms in cardiac tissue: Ca(V)3.1 and Ca(V)3.2; also, Ca(V)1.3 is the main isomer of I CaL in the heart; Mesirca et al. expressed that severe forms of congenital bradycardia and atrioventricular block in pediatric patients are associated with functional loss of Ca(V)3.1 isomer of I CaT receptors; however, all types of VGCC, Ca(v)1.2, mediate excitation-contraction coupling which is a crucial process in contractile function and discussed later in the chapter (Sobie et al. 2006; Ono and Iijima 2010; Mesirca et al. 2014, 2015).
- 3.
In cardiac pacemaker cells, the slope of AP in phase 4 is not as flat as myocardial cells; instead it is upward, known as prepotential steep phase, and is mainly due to three main factors:
Funny current: I(f)
Ca2+ influx by I CaT
K+ efflux by potassium channel (I K)
- 4.
On the other hand, the main role of I CaL is in phase 0; in fact, in pacemaker cells (SA node and AV node), I CaL exerts its main role in phase 0, and “Ca2+ influx” in pacemaker cells replaces “Na+ influx” of myocardial cells.
- 5.
Phase 1 (i.e., short-term repolarization) is nearly deleted or, better to say, integrated in phases 2 and 3.
- 6.
Table 3.2
Action potential in cardiac pacemaker cells


Fig. 3.4
Action potential (AP) in cardiac pacemaker cells

Fig. 3.5
The difference between action potential phases in normal and pacemaker cells
There is another important concept in action potential of many cells including myocardial cells and that is “refractory period” which is the time interval during which there is no response to new impulse or the response could be sluggish: absolute refractory period or relative refractory period, respectively. Though described as one of the basic properties of currents alongside the cell membrane, some studies strongly believe that post-repolarization refractoriness plays a protective role against “reentrant” mechanisms of arrhythmias, especially in preventing atrial fibrillation.
In all cells having refractory period, the time interval for refractoriness is proportional to the time duration of action potential, and it is primarily dependent on the duration of phase 2 “plateau,” so:
In atrial cells, the refractory period is usually shorter (about 0.15 s).
In ventricular cells it is about 0.25–0.3 s.
What is the underlying mechanism for automaticity of pacemaker cells? Three theories are proposed: M-clock theory, Ca 2+ clock theory, and coupled clock theory, described here in brief:
M-clock theory
This theory, known as membrane clock (or just briefly M-clock), implicates that ion channels and ion transporters located on myocardial cell membrane, their inward and outward currents, and their associated channels (i.e., mainly L-type Ca2+ channels, K+ outflux channels, Na+/Ca2+ exchanger “NCX,” Na+/K+ ATPase, and funny currents) are the main generators of action potentials needed for pacemaker activity; this theory involves mainly the role of sarcolemma-related structures.
Ca2+ clock theory
This theory implies that Ca2+ is restored in the sarcoplasmic reticulum, the related Ca2+ pumps (sarcoplasmic/endoplasmic Ca2+ ATPase, “SERCA”), Ca2+ channels including ryanodine receptor family (RYRs), and some members of the protein kinase family oscillate rhythmically and periodically to produce the pacemaker automaticity.
Coupled clock theory
Though none of the two above theories are frankly dominant over the other theories, both cooperate to produce the pacemaker function in the heart having important role in impulse generation; however, their cooperation with other proteins like protein kinase A (PKA) or Ca2+/calmodulin-dependent protein kinase II (CaMK II) is the basis for the model known as “coupled clock theory” being the most recent proposed theory for impulse generation and automaticity in the heart especially in SA node; this theory implicates the wide interactions between cell membrane-related mechanisms and intracellular mechanisms leading to impulse generation (Maltsev et al. 2006, 2014; Sobie et al. 2006; Maltsev and Lakatta 2007, 2012; Mangoni and Nargeot 2008; Lakatta et al. 2010; Yaniv et al. 2012, 2015).
Excitation-Contraction Coupling: ECC
ECC is the “linkage” phenomenon which translates the electrical activities of the heart to the mechanical activities; hence, it is the “electromechanical interface.” Nearly 50 years ago, this concept was proposed, and since then, a great bulk of researches have been done on this topic (Quinn et al. 2014). The terminal and eventual goal in ECC is the process of Ca2+ cycling leading to rhythmic initiation and termination of myocardial contraction. ECC has three main parts:
Ca2+
Functioning organelles of ECC
ECC-modulating mechanisms
Calcium Homeostasis
Calcium homeostasis should be discussed at first since Ca2+ homeostasis has a central role in ECC. As a matter of fact, Ca2+ homeostasis is one of the most important cellular processes in all cells including myocardial cells. Intracellular Ca2+ is not only a second messenger, but in myocytes, it has other roles; the dual-phase pattern of Ca 2+ cycling (i.e., Ca2+ release and Ca2+ reuptake) is the basis for lifelong contraction and relaxation of the heart; needless to say, many intracellular proteins are involved in Ca2+ homeostasis; on the other hand, many intracellular interactions of the heart are dependent on Ca2+ homeostasis, including:
Electrical function (with Ca2+ influx and efflux as integral parts of action potential)
Mechanical activities (with Ca2+ surge in systole and Ca2+ reuptake in diastole)
Cell energetics, especially inside the mitochondria
Buffering capacity of the cells for stress control
Apoptosis and cell death-dependent mechanisms
The sarcoplasmic reticulum (SR) is the main source of intracellular Ca2+ storage; however, in “pediatric heart” the amount of Ca2+ in SR is not as abundant as the SR of adult heart; so, the myocardium of the neonates, infants, and children is highly dependent on extracellular levels of Ca2+ for functioning normally, especially when considering the fact that the calcium transport system in the myocardium of infants and children is immature. These unique features of Ca2+ homeostasis in pediatric heart is among the main causes for reduced myocardial in this age group compared to the normal healthy adult (Bers 2002; Maier et al. 2005; Gustafsson and Gottlieb 2009; Asp et al. 2013).
The excitation-contraction coupling (ECC) involves a number of ongoing and interrelated events to act as a machine to promote cardiac function. The following steps occurring in sequential cascade constitute the ECC:
- 1.
I CaL or dihydropyridine receptor (DHPR) opens for initial primary Ca2+ influx, (Ca2+ trigger) from extracellular fluid (ECF).
- 2.
The primary “Ca2+ trigger” causes release of huge Ca2+ amounts from the junctional sarcoplasmic reticulum through ryanodine receptor-2 and other intracellular calcium reservoirs; in fact, this small primary amount of Ca2+ sparks the release of next huge amounts of Ca2+; this phenomenon is called calcium-induced calcium release (CICR); among many interesting features of CICR is that in some pathologies, CICR instability contributes to cardiac arrhythmias (Fabiato and Fabiato 1977, 1978; Fabiato 1983; Bers 2002).
- 3.
CIRC causes huge Ca2+ release; then, Ca2+ interacts with contractile elements of the myocardial cells, starting myocardial contraction.
- 4.
Immediately afterward, Ca2+ recycling from the cytosol back to the sarcoplasmic reticulum (SR) happens; this process is the main mechanism for Ca2+ reuptake and is done mainly by an ATP-dependent protein called sarco-/endoplasmic reticulum Ca2+ transport ATPase (abbreviated as SERCA) functioning as a pump to recollect Ca2+; more than ten isoforms of SERCA have been recognized in different tissues; however, the primary isoform recognized in myocardial cells is SERCA2a; however, SERCA2a activity is not limitless and is modulated by another protein called phospholamban (PLB); PLB blocks SERCA2a through biochemical and structural transitions of its molecule (Sobie et al. 2006; Williams et al. 2010).
- 5.
Besides SERCA2a, the remainder of Ca2+ is returned to ECF by NCX.
So, these three main proteins interact together in handling of Ca2+ homeostasis:
First: SERCA2a mediates Ca2+ reuptake and starts myocardial relaxation; in diastolic dysfunction, SERCA2a is one of the most important therapeutic targets which is the goal for novel therapies aimed to augment SERCA2a or phosphorylation of PLB (i.e., inactivation of PLB; also see next paragraph) (Shareef et al. 2014). In one study, Pavlovic et al. demonstrated that the “PLB/SERCA ratio was significantly reduced in atrial myocardial tissue of pediatric patients with volume overload” (Pavlovic et al. 2005).
Second: PLB modulates SERCA2a activity and so stops myocardial relaxation and, indirectly, stops diastole; also, PLB determines cardiac response to beta-1 adrenergic stimulation. When cardiac muscle is stimulated through β-1 receptor, increased cAMP ensues leading to increased activity of protein kinase A (PKA); increased phosphorylation of PLB on serine-16 as the result of increased PKA activity results in relieving PLB inhibitory effect on SERCA2a activity; the final outcome of this story is increased Ca2+ pumping to the SR and accelerated reuptake of Ca2+ from cytosol; the physiologic picture of this cascade would be speeding up the myocardial relaxation and shortening diastole; augmented PLB phosphorylation could be a potential future therapy for diastolic dysfunction (Frank et al. 2003; Asp et al. 2013; Espinoza-Fonseca et al. 2015).
Third: Sarcolipin (SLN) is another cytosol protein that regulates Ca2+ homeostasis mainly in atrial myocytes somewhat similar to PLB in ventricular myocytes; though SLN and PLB are not the same regarding their structure, function, and anatomic site of action, their final function is to inhibit SERCA2a activity in order to terminate SERCA2a role in Ca2+ reuptake; decreased SLN in atrial myocytes is associated with augmented activity of SERCA2a in atrial myocytes and SR Ca2+ overload which is associated with atrial fibrillation and atrial remodeling (Bhupathy et al. 2007; Periasamy et al. 2008; Xie et al. 2012).
There are a number of congenital heart diseases titled under “conotruncal defects” resulting from altered neural crest migration which include tetralogy of Fallot (TOF), transposition of great arteries, persistent truncus arteriosus, double-outlet right ventricle, interrupted aortic arch, and other anomalies of aortic arch; in these congenital cardiac anomalies, neural crest impairment leads to important defects in SR, especially impaired RYR-2, impaired ECC, and defective I CaL; also, in some of these anomalies (mainly in TOF), impaired function of PLB and SLN is seen as a prominent defect in the myocardial cytosol (Vittorini et al. 2007).
Other proteins like calmodulin (calcium-modulated protein) and calsequestrin are discussed here.
There is another protein named calmodulin-dependent protein kinase II (CaMK II) which also could control and modulate SERCA2a (i.e., somewhat similar to PLB) (Tables 3.3 and 3.4).
Table 3.3
A summary of ECC
Event
Protein or channel in-charge
The main phenomenon
Result
1.
Initial Ca2+ influx (Ca2+ entry to the cell)
I CaL DHPR
DHPR channel opens
Triggers CICR (opening of RyR-2)
2.
Ca2+ release (CICR) due to opening of RyR-2
RyR-2
Channel opens and huge Ca2+ is released from SR
Starts myocardial contraction
3.
Ca2+ recycling, from cytosol back to SR, i.e., Ca2+ reuptake and Ca2+ efflux
SERCA2a (mainly) and NCX
Recollection of Ca2+ from cytosol
Starts myocardial relaxation
4.
Modulation of SERCA2a
PLB
Stopping Ca2+ reuptake by SERCA2a
Stops myocardial relaxation; the next contraction could now start
Table 3.4
A summary of the composing aspects of ECC and their related items
1.
Functioning organelles of ECC
Cell membrane
Thick and thin filaments
T tubules
Sarcoplasmic reticulum
2.
Calcium ion (Ca2+)
Ca2+ influx to the cardiomyocytes (by L-type Ca2+ channels in systole)
Ca2+ release inside the cell (by RyR in systole)
Ca2+ efflux from the cardiomyocytes (by NCX in diastole)
Ca2+ reuptake from the cell (by SERCA in diastole)
3.
Controllers of ECC
Ryanodine receptor (RyR) family
Dihydropyridine receptor (DHPR)
Calmodulin
Functioning Organelles of ECC
Functioning organelles of ECC are cell membrane, thick and thin filaments, T tubules, and sarcoplasmic reticulum:
- 1.
The myocardial cell membrane or sarcolemma is the main player for both parts of ECC, i.e., the electrical phase of ECC (which includes creation of different phases of action potential) and the mechanical phase of ECC (which is primarily through Ca2+ trigger).
- 2.
Thick and thin filaments.
- 3.
Transverse tubules (T tubules).
- 4.
Sarcoplasmic reticulum (SR), being divided to longitudinal SR (LSR) and junctional SR (JSR), acts as the main intracellular Ca2+ reservoir which releases and reuptakes Ca2+ per needed into and out of the cytosol; RyR-2 and SERCA are the main Ca2+ releasing and reuptaking pump located on JSR, respectively.
ECC-Modulating Mechanisms
There are a number of modulators which balance the different interactions in ECC (Yang et al. 2011; Asghari et al. 2014; Brunet et al. 2015; Motloch et al. 2016):
PKC modulates ECC.
All types of VGCC, Ca(v)1.2, mediate ECC.
There are a number of uncoupling proteins (UCPs), mainly located in the inner membrane of cardiac mitochondria; they belong to a superfamily of mitochondrial ion transporters, and they modulate intracellular Ca2+; so, they modulate ECC by modulating mitochondrial Ca2+ uptake.
The micro-architecture of the myocardial tissue has many aspects which have modulating role on ECC which are under further assessments.
Mechanical (Contractile) Function of the Myocardium
The contractile function of the myocardium is the specific duty performed by two syncytia: atrial and ventricular. These two syncytia are composed of a huge number of units called sarcomere. Each sarcomere is defined as a specialized part of the myocardial muscle located between two Z lines; the main ingredients of sarcomere are:
Contractile proteins which are responsible for myocardial contraction (actin and myosin) and the backbone (such as titin); these are arranged in a delicate structure between two Z lines and are discussed later.
Regulatory proteins which control the cyclic contraction-relaxation phases; these are mainly troponin, tropomyosin, tropomodulin, and myosin-binding protein C, which activate and modulate sarcomere functions. The contractile elements of the myocardium could be divided as shown in Table 3.5.
Table 3.5
Categories of myocardial filaments
Contractile proteins
Modulatory proteins
Thick filament
Myosin
Titin
Myosin-binding protein C (MBPC)
Thin filament
Actin
Tropomyosin
Troponin (TnC, TnT, TnI)
Tropomodulin
Inside each of the sarcomere, actin and myosin interactions lead to “Ca2+-triggered crossbridges” between actin and myosin leading to contraction; this contraction is the third stage after electrochemical action potential translated via “ECC” pathway to contraction; so, the more crossbridges, the more contractile force. After each contraction, troponin I (TnI) detaches actin and myosin from each other; hence crossbridge sites (active sites) are disappeared. Crossbridges need great amounts of fuel supplied as sarcomere ATP reservoirs; both attachment and separation of crossbridges are energy consuming. This is the basis for cardiac systolic contraction and diastolic relaxation.
Although there are many similarities in the function and structure of the contractile tissue between healthy adult heart and the pediatric hearth, we should always keep in mind that the texture of the myocardium in pediatric heart is organized in such a way that only about 50 % of the tissue is composed of contractile elements, while the rest 50 % of the tissue is composed of noncontractile elements, including mitochondria and large nuclei. This unique structural feature has a number of specificities:
- 1.
In the pediatric myocardium, the percentage of contractile mass in the total mass of myocardium is less than the adult myocardium; on the other hand, decreased contractile mass leads to decreased compliance of the ventricular muscle (compared to the adult healthy heart); this is one of the main reasons why the myocardial contractile reserve is significantly reduced compared to the healthy adult myocardium.
- 2.
Due to decreased compliance of the LV, the filling pressures (compared with adult healthy myocardium) are higher, and preload augmentation is limited to just 1–7 mmHg.
- 3.
The neonatal myocardium is hence highly dependent on the resting tone of β-adrenergic stimulation; in clinical practice, we see very high sensitivity of the neonatal myocardium to even low doses of β-blockers, and on the other side, the response to β-agonists is not so much exaggerated as adult healthy myocardium.
Here, we first describe the main ingredients of each sarcomere, and then we will describe their assembly including Z disc (Z line), M band, I band, and A band and how thin and thick filaments are integrated in the framework of these structures in sarcomere.
Thick Filament
Thick filament
Thick filament is composed mainly of two contractile proteins (myosin and titin) and one modulatory protein (myosin-binding protein C or briefly MBPC).
Myosin has a structured framework. First of all, the 15 nanometer myosin rods are composed of the following elements:
One myosin heavy chain (1 MHC)
Two myosin light chains (2 MLCs)
Then, one MHC plus two MLCs compose a single myosin strand (MS); so, we will have:
Afterward, two MSs woven together produce one myosin molecule (MM); in other words, each MM is composed of two MSs:
However, each MM resembles a “golf club” which has two functional domains: the four MLCs in the head region and the tail region of MM which is like the handle of the golf club (Fig. 3.1). Ca2+ trigger causes the head of MM to cross bridge with actin leading contractions. Also, TnI inhibits the crossbridges at the end of each interaction to detach actin from myosin head.


About 300 MMs collectively form together in a parallel fashion forming myosin rod (MR) which resembles “a bundle of golf clubs” collected together while their heads protrude out of the bundle and their bodies and handles are attached together (Fig. 3.6).


Fig. 3.6
Schematic presentation of a myosin molecule
Titin is the most frequent filament after actin and myosin; “the largest protein known to date,” titin is a very giant filament extending from each side of the Z line toward the M line; it has been simply named as the “bidirectional molecular spring” of the cardiac sarcomere; in other words, during diastole, the extensible properties of titin help myocardial tissue to preserve its primary configuration, returning to the primary size of the heart; in this way, titin acts as a “passive force generator” in diastole; on the other hand, titin plays its role in restoration of sarcomere length when it is shorter than its slack length. Besides, titin has attachments with actin-myosin crossbridge sites through myosin-binding protein C (MBPC). This is why titin has a very strategic role in cardiac sarcomere to keep it stable during contraction and relaxation (Pyle and Solaro 2004; Solaro 2005; Kobirumaki-Shimozawa et al. 2014).
Titin has a specific structure which helps create its elastic role. Functionally speaking, titin has two segments:
The extensible segment anchored to each side of the Z line located in the I band domain; this is the N terminal of titin.
The non-extensible segment located in the A band; the end of C terminal is bound to the thick filament.
On the other hand, if we want to explain titin based on a structural classification of the molecule, it has two parts:
Immunoglobulin-like segment.
PEVK segment abbreviated for the four amino acids: proline (P), glutamate (E), valine (V), and lysine (K); these amino acids compose about 75 % of the PEVK segment.
The role of titin in “fine-tune passive myocardial stiffness” and “diastolic function and diastolic force generation” is highly dependent on phosphorylation of titin molecule by calcium-/calmodulin-dependent protein kinase II delta (CaMKIIδ); those regions of titin located in “I band” are important in phosphorylation mechanisms. Impairment in CaMKII-dependent phosphorylation of titin is considered as a major etiology for diastolic dysfunction. Mutations in those genes related to titin are often considered as the etiologic factor for dilated cardiomyopathy, since titin disruption ends to impairment of structure of the heart and its elasticity (LeWinter et al. 2007; Nishikawa et al. 2012; Hamdani et al. 2013a, b; Hidalgo et al. 2013; Kotter et al. 2013; Rain et al. 2014; McNally et al. 2015; Zile et al. 2015).
Titin stiffness could lead to RV diastolic dysfunction in pulmonary hypertension patients, of course in association with factors like reduced phosphorylation of cTnI and altered phosphorylation of Ca2+ (Rain et al. 2014).
Also, in heart failure patients, impaired phosphorylation of the thick filament protein myosin-binding protein C (cMyBP-C) has a main role especially in patients with diagnosis of familial hypertrophic cardiomyopathy due to mutations in MYBPC3 (Kuster et al. 2012).
Thin Filament
Thin filament is composed of actin (mainly in the form of filamentous actin “F actin”), tropomyosin (TM), and troponin complex (Tc); if we want to compare the number of molecules for each protein in the thin filament, then we will have one F actin, two TM, and two Tc in each imaginary unit of thin filament.
Actin
Actin is made of F actin; so, F actin is the backbone of the thin filament, while TM is located in the groove of F actin strand; however, Tc is located at defined and regular intervals along F actin. Each molecule of F actin is composed of 13 subunits called globular actin “G actin,” which twist to form a 360 degree turn in F actin strand. In cardiac sarcomere, F actin is alpha subtype.
Tropomyosin (TM)
Tropomyosin is a right-handed helical coiled coil and inhibitory protein located on F actin. Two adjacent TM molecules attach together and turn round each other; then, this coiled filament (composed of two turned TM molecules) twists once more while being attached to actin filament; so, it is termed “the prototype coiled coil” protein. Each TM molecule is in contact with seven subsequent G actin molecules; so their molecular ratio is “Tm 1:G actin 7.” The end of one TM molecule is attached to the head of the next TM molecule; this attachment, called head-to-tail overlap of TM, has about eight amino acids. This head-to-tail interaction of each two subsequent TM molecules is of the most determining factors modulating the function of thin filament. Of course, recent studies have demonstrated that this description is somewhat a simplified picture of the real structure of TM (Kobayashi et al. 2008; Nevzorov and Levitsky 2011).
TM is under the control of two parts of troponin complex: troponin T (TnT) and troponin I (TnI); in cardiac muscle, Ca2+ attachment to troponin C (TnC) causes TM to be detached from the actin-myosin crossbridge site; this is exactly the point which is inhibited by TM; in other words, Ca2+ inhibits TM; then, this “inhibition” exposes the “crossbridge site” to myosin molecule; this is exactly the myosin binding site on actin molecule known as crossbridge site or active site; in this process, when TM is pushed far from crossbridge sites, myosin crossbridge finds the opportunity to directly attach to this critical point, i.e., the crossbridge; and these interactions lead to sarcomere contraction. At the end of the contraction, when Ca2+ concentration in sarcomere falls, troponin I (TnI) augments the role of TM, in such a way that again TM becomes closer to crossbridge sites, attaches to crossbridge, and pushes myosin away from crossbridge; the final outcome is inhibition of contraction. In this process, the two most important modulators for sensitivity of sarcomere filaments to Ca2+ are the sarcomere length (i.e., Frank-Starling relationship) and the role of protein phosphorylation in sarcomere which causes posttranslational modification of these proteins; the reader can find detailed discussion in a number of reviews published in the last years (Hitchcock-DeGregori 2008; Kobayashi et al. 2008; Jagatheesan et al. 2010; Bai et al. 2013).
There are two specific inherited diseases involving impaired function of TM in Ca2+ attachment: dilated cardiomyopathy (DCM) and hypertrophic cardiomyopathy (HCM); both are due to “missense mutations” in genes coding TM. In summary, in DCM, the mutation causes decreased sensitivity of TM to Ca2+ binding, while in HCM, the mutation ends to increased sensitivity of TM to Ca2+ (Bai et al. 2013; Redwood and Robinson 2013; Kalyva et al. 2014) (Fig. 3.7).


Fig. 3.7
Schematic presentation of a thin filament (also see Fig. 3.8a): each helix of F actin contains two strands of G actin each containing 13 G actin molecules (blue and red globes) and myosin binding sites which expose actin for crossbridging with myosin and located on each G actin (demonstrated as a black dot); troponin complex (green knobs) and tropomyosin double-strand coiled coils as (yellow threads)
Troponin complex (Tn)
Troponin complex is composed of three subsegments: troponin C (TnC), troponin I (TnI), and troponin T (TnT); TnI inhibits the crossbridge by inhibiting the actin-myosin-TM interactions, while TnC activates muscle contraction by “inhibiting the inhibitory role of TnI”; TnT is the modulator for troponin activity. Troponin complex is not just a simple attachment of three proteins; instead, the conformational interactions between these three components are mandatory for the activity of troponin complex.
Troponin C (TnC)
This protein acts as a “Ca2+ sensor” which senses and regulates the sequential events involved in initiation and control of contraction inside the sarcomere. The shape of TnC is much similar a dumbbell; two heads of the dumbbell are the N terminus and the C terminus of TnC molecule.
There are two Ca2+ binding sites (CBS) on C terminus (CBS III and CBS IV); Ca2+ is attached firmly to both of them; so none of them is involved in controlling Ca2+-dependent muscular contractions; however, these two Ca2+ binding sites (i.e., CBS III and IV) are attached to TnC and TnI. On the other hand, there are two other Ca2+ binding sites on the N terminus of TnC: CBS I and CBS II; however, CBS I cannot attach to Ca2+ firmly, while CBS II is the only and most important CBS that attaches to Ca2+ and performs the regulatory and modification roles of TnC in muscle contraction. Attachment of Ca2+ to CBS II on N terminus of TnC causes structural and functional changes in both “actin-TM” complex and troponin complex; these changes cause activation of the thin filament. TnC mutations are involved in both HCM and DCM (Marston and Redwood 2003; Kobayashi et al. 2008; Kekenes-Huskey et al. 2012; Craig et al. 2014; Kalyva et al. 2014; Kobirumaki-Shimozawa et al. 2014; Shi et al. 2015).
Troponin I (TnI)
This part of troponin has an inhibitory role; not only TnI alone inhibits actin, but also, when combined with TM, the TnI-TM complex acts as a potent inhibitor of actin-myosin interaction and so prevents generation of forceful contractions. Some genetic disorders in TnI cause hypertrophic cardiomyopathy (HCM) or restrictive cardiomyopathy (RCM) because of impairment in inhibitory effects of TnI in diastole. The interested reader is addressed to detailed references for TnI (Chang et al. 2008; Kobayashi et al. 2008; Ohtsuki and Morimoto 2008; Solaro et al. 2008; 2013).
Troponin T (TnT)
TnT is an integrating component of thin filament and connects TM to troponin complex. TnT attaches to the “head-to-tail” segment of two subsequent TM segments on one hand and to the Z disc on the other hand; this protein has a cooperative role in thin filament activation through its modulatory role on TM activity. To explain more, the inhibition of TnI would be relieved without any need of Ca2+ if there was no TnT; but in the presence of TnT, Ca2+ is mandatory for TnC activity in order to lift the inhibitory role of TnI. TnT is composed of two subfragments: TnT1 (N terminal) and TnT2 (C terminal). TnT1 binds strongly to tropomyosin, while TnT2 has the important role in Ca2+ regulation; the function of TnT on Ca2+ regulation would be impaired without TnT2. The majority of the mutations leading to DCM are related to TnT though TnI and TnC also have mutations leading to DCM. Also, some mutations in TnT lead to HCM (Chang et al. 2008; Kobayashi et al. 2008; Ohtsuki and Morimoto 2008).
A main functional part of interactions between these proteins is the crossbridging phenomenon, a basic phenomenon with its integral role in myocardial contraction, which is based on continuous interactions between the thick and thin filaments.
Z Disc, I Band, A Band, and M Line
Z disc, I band, A band, and M line (the following components are demonstrated in Fig. 3.8a, b): Sarcomeres are cylindrical repeating units; the margin of each sarcomere is Z disc or Z line.


Fig. 3.8
(a) Schematic presentation of microscopic structure of a sarcomere from a wide view (also, see Fig. 3.7). (b) Schematic presentation of microscopic structure of a sarcomere (compare each part with its compared part in Section A) (Figure b is modified from Dabbagh (2014). Published with kind permission from © Springer, 2014. All Rights Reserved)
Z in Z discs stands for Zuckung, a German name meaning twitch; Z discs are three-dimensional structures about 100 nm (0.1 μ) composing the margins of each sarcomere; in fact, Z discs are the border of two neighboring sarcomeres, and they divide the sarcomeres from each other. However, each Z disc is a complex of many proteins including those located at Z disc and those attached to Z disc.
Functionally, Z discs have the main following roles:
Producing mechanical stability in each contraction and relaxation, since Z discs are the anchoring site of many sarcomere proteins and, also, thin filament and titin
Transmission and transduction of signal between two adjacent sarcomeres
Each Z disc is surrounded in each side by I band, which is the dark band adjacent to the Z disc; in fact each I band spans thin filaments of the two adjacent sarcomeres with Z disc located in its center.
There is a pivotal band for the thick filaments called M band; these bands are in the Middle of the sarcomere, so M band. Both Z discs and M bands are transversally oriented multi-protein scaffolds (Stehle et al. 2009). On either side of each M band, thick filaments compose the “A band.” In fact, A band is the band on each side of the M band which is the region of thick filaments; the lateral border of A band contains the terminal segment of the thin filaments which, together with thick filaments, slide into each other during contraction and come out during relaxation (Tskhovrebova and Trinick 2010).
One A band in the middle plus two “half I bands” in each side compose the span of one sarcomere which is between two Z discs.
On the other side, the specific segment of the A band located in the center of A band which is devoid of thin filaments is called “H band” (Solaro 2005; Kobayashi et al. 2008).
In summary, the attachment of filaments of sarcomeres is as follows (Stehle et al. 2009):
Thick filaments are anchored just at its midpoint to the M line; then, each thick filament spans in each of the two lateral sides toward the Z discs; however, it does not attach Z disc.
Thin filaments are attached and anchored to Z lines and extend from Z line toward the midpoint of the sarcomere, i.e., the M line; again, thin filaments do not attach to M line.
Titin, the huge protein of the thick filament, is attached on one side to Z disc and on the other side to M band; so, titin is spread all along the sarcomere from the Z disc on one hand to the M band of the sarcomere on the other hand; also, there is alpha actinin, a specific protein located in Z line, connecting thin filament with titin (Anderson and Granzier 2012).
Novel Therapeutic Agents Against Heart Failure
Based on the protein molecules and the protein architecture involved in myocardial contraction, a wide array of novel therapeutic agents are under assay which is briefed here:
Omecamtiv mecarbil, a myosin activator agent, enables myosin to be attached much more “firmly” to actin to produce more forceful myocardial contraction; this drug acts directly on sarcomere, without any perturbation in myocardial Ca2+ homeostasis inside the cell; in fact, omecamtiv mecarbil increases the rate of actin-dependent release of phosphate, resulting in increased actin-attached myosin heads, causing increased number of active, force-generating myosin heads during systole and also increased effectiveness of myosin crossbridge formation and duration; so, systolic ejection time increases without any unwanted effects on the left ventricular filling pressure or any unwanted increase in myocardial contraction velocity; the drug seems to be a good pharmacologic agent for systolic heart failure; however, it is not yet available for clinical use (Cleland et al. 2011; Malik and Morgan 2011; Teerlink et al. 2011; Aronson and Krum 2012; Garg and Frishman 2013).
Istaroxime has a dual function; first, it inhibits sarcolemmal Na+/K+ ATPase to increase intracellular Na + concentration and to decrease Ca2+ efflux through NCX, increasing intracellular Ca2+ concentration; second, istaroxime stimulates SERCA2a to augment lusitropic properties of the myocardium and decreases the chance for arrhythmias; it also protects the heart through stabilization of SERCA2a (George et al. 2014; Ahmad et al. 2015).
In diastole, Ca2+ overload happens which is either due to decreased rate of Ca2+ reuptake or increased sensitivity of myocardium to Ca2+. Novel therapies have been proposed to treat diastolic dysfunction through augmentation of SERCA2a or partial inhibition of PLB (Kawase and Hajjar 2008; Teerlink et al. 2009; Kawamura et al. 2010; Asp et al. 2013).
Control Mechanisms of Cardiac Function
Autonomic Control of the Heart
The autonomic nervous system (ANS) as a portion of the peripheral nervous system continuously controls cardiovascular functions.
The ANS, on the other hand, is regulated by other centers such as the brain stem and hypothalamus.
The sympathetic and parasympathetic systems are the main component of ANS.
The preganglionic nerves of sympathetic system originate from T1-L2 segment of the spinal cord and enter the sympathetic chain ganglia located along the side of the viscera column (i.e., paravertebral ganglia). Postganglionic fibers of the cardiac sympathetic originate from the stellate ganglia and extend to different parts of the heart (SA and AV nodes, atria, and ventricles).
The long preganglionic nerves of cardiac parasympathetic system originate in the dorsal motor nucleus of the tenth cranial nerves (left and right vagi) or in the nucleus ambiguous. The short postganglionic nerves lie on epicardial surface and within the atrial and ventricular walls. There is no equal vagi distribution to different cardiac structures, because the vagi mainly affect SA and AV nodes and there is a slight distribution for atria and ventricles.
In the newborn heart, the number of α-adrenergic receptors is reduced; this is among the factors that limit the function of the left ventricle; also, the level of the circulating catecholamines increases dramatically to compensate for the limited function of the heart, an important fact that should be considered when anesthetizing a child or newborn especially for cardiac procedures; it is logic to avoid suppressing the sympathetic tone of the body in order to prevent decreased cardiac output.
Since the right and left sides of the embryonic structures are the sites for developing the SA and AV nodes, respectively, the SA node receives its ANS branches mainly from the right vagus and right stellate ganglion, and the AV node takes its branches mostly from the left vagus and left stellate ganglion. Sympathetic β-receptors are epicardial and parasympathetic muscarinic receptors are endocardial (Gordan et al. 2015).
Sympathetic and Parasympathetic Receptors
There are different subtypes of α- (α1A, α1B, and α1D) and β (β1and β2)-sympathetic (or adrenergic) receptors in the heart.
Although, the number of α1-receptors is less than β-receptors, activation of α1-receptors leads to increased ventricular contraction, sensitivity of contractile myofilaments to calcium ions, and cardiac hypertrophy. Activation of the α1-receptor as a Gq/11-coupled receptor by stimulating phospholipase-C (PLC) leads to enhanced intracellular inositol triphosphate (IP3) and diacylglycerol (DAG) concentration.
The β1-adrenergic receptors are expressed on the different portions of the heart such as the SA node, AV node, and atrial and ventricular cardiomyocytes. The activation of β1-receptors increases heart rate and augments myocardial contractility by increasing of the intracellular calcium concentrations through calcium currents from extracellular fluid, and the sarcoplasmic reticulum (SR) stores and enhances the AV node conduction velocity. The β1-adrenoceptor is a Gs-protein-coupled receptor, and activation of αs (as a subunit of G-protein) by stimulation of adenylyl cyclase increases the cAMP production and raises protein kinase A (PKA) activity. Although, the number of the β2-receptors subtype is less than the β1-receptor, the effects of both subtypes are similar to each other.
The M2 subtype of muscarinic receptors is dominant receptor for parasympathetic nervous system in the heart. This receptor is coupled to the Gi-protein, and activation of αi by inhibition of adenylyl cyclase reduces cAMP production and lessens PKA activity. In addition, the βγ portion of Gi-protein activates acetylcholine-sensitive potassium channels (IKAch) and creates hyperpolarization (Thomas 2011).
Functions of the ANS in the Heart
The ANS has a prominent effect on the different features of the heart due to its capacity to modify heart rate (chronotropy), contractility (inotropy), conduction velocity (dromotropy), rate of relaxation (lusitropy), and degree of excitability (bathmotropy).
Chronotropy changes are created via changes in steepness of pacemaker potential in SA node; inotropy is regulated by modulation of myocardial force generation through different mechanisms; dromotropy is altered by changes in the speed of impulse conduction in the AV node; lusitropy is controlled by the manners that return the heart muscle to its initial relaxed condition after each muscle contraction, and bathmotropy is modified by changes in the threshold of heart excitation. The different effects of the β1– and M2 receptor activation on the heart has been summarized in Table 3.6 (Myslivecek and Trojan 2003).
Table 3.6
Myocardial receptors characterized by their properties
Receptor type | Inotropy | Chronotropy | Dromotropy | Lusitropy | Bathmotropy |
---|---|---|---|---|---|
β1 | + | + | + | + | + |
M2 | − | − | − | − | − |
Inotropic Effects of the β1– and M2 Receptor Activation
Intracellular rise of free Ca2+ concentration is a fundamental factor for initiating myocardial contraction, and for this, two important Ca2+ sources play a part: (1) extracellular fluid (ECF) and (2) sarcoplasmic reticulum (SR). Nearly, 20 % of needed Ca2+ is provided from ECF and the remaining (80 %) from SR. During the plateau phase of the action potential, Ca2+ enters the muscle fiber from ECF via L-type Ca2+ channels for generating the great release of Ca2+ from the SR through ryanodine channel in the CICR phenomenon described earlier in this chapter.
There are many reasons for positive inotropic effect of β1-adrenoceptor stimulation by released catecholamines from postganglionic neuron of sympathetic system (norepinephrine) and the adrenal gland (epinephrine):
- 1.
Increase in the PKA activity via phosphorylation of L-type Ca2+ channel leads to increase in the Ca2+ influx.
- 2.
Increase in the Ca2+ influx leads to a greater release of Ca2+ from the SR, and this action reinforces the intracellular concentration of Ca2+.
- 3.
Catecholamines provide further Ca2+ stores in the SR for next contraction by increase in activity of SERCA and further Ca2+ pumping into the SR at the end of previous contraction. In addition, enhanced Ca2+ influx through L-type Ca2+ channels leads to increase in Ca2+ availability for more storage in the SR.
- 4.
Sensitivity of troponin C for Ca2+ is increased, and this action facilitates the sliding of actin and myosin filaments for contraction achievement.
As mentioned earlier, stimulation of the vagus nerve has a slight direct effect on myocardial contractility due to poor distribution of parasympathetic on the atria and ventricles. On the other hand, severe stimulation of the vagus nerve has a significant inhibitory effect on myocardial contractility mainly by reducing heart rate.
Chronotropic Effects of the β1– and M2 Receptor Activation
Activation of Gs-protein-coupled β1-adrenoceptor by pharmaceutical agents like epinephrine and norepinephrine enhances the heart rate through two mechanisms:
- 1.
By increasing IF (Ih) and ICa in the SA node which leads to increase in steepness of pacemaker potential of action potential
- 2.
By increasing ICa, which causes more negative threshold of the action potential
Both of the above mechanisms accelerate the generation of the next action potential and shorten the interval between two consecutive action potentials.
On the other hand, in contrary to β1-adrenoceptor, activation of Gi-protein-coupled M2 receptor by acetylcholine (Ach) slows the pacemaker activity of the SA node through three mechanisms, leading to decreased heart rate:
- 1.
Acetylcholine decreases the steepness of pacemaker potential by reducing IF and ICa.
- 2.
Activated βγ portion of Gi-protein opens acetylcholine-sensitive potassium channels (IKAch) and increases outward potassium current; this effect produces “a hyperpolarization state” in the SA node.
- 3.
Reduction of ICa changes the threshold of action potential to more positive level.
All these three mechanisms decrease the speed of action potential generation, finally reducing heart rate (Chemla et al. 2000).
Lusitropic Effects of the β1-Receptor Activation
Catecholamines enhance cardiac relaxation by stimulation of β1-adrenoceptor. After binding of catecholamines to these receptors, protein kinase A (PKA) is activated. This activated enzyme can phosphorylate some proteins in the cardiomyocytes such as phospholamban which is a regulator of ion transport and a major substrate for PKA. Phospholamban reduces the uptake of Ca2+ into the sarcoplasmic reticulum by inhibiting SERCA and this effect creates delay for starting myocardial relaxation after each contraction. Phosphorylation of phospholamban by PKA declines the inhibitory effect of phospholamban on SERCA and leads to an increase in reuptake of Ca2+ into the SERCA (as described earlier in this chapter). Finally, reduction of intracellular Ca2+ level creates the earlier relaxation.
Phosphorylation of the troponin I is another action of PKA in order to augment myocardial relaxation; this effect reduces affinity of troponin C for Ca2+ binding and provides relaxation condition for cardiomyocytes.
Developmental Changes in Fetal Cardiac Contractile System
In this section, a number of developmental changes in cardiovascular system are discussed. Of course, in the first sections of this chapter, “evolutional transition in cardiac physiology” is discussed in detail and is not repeated here.
Developmental Changes in Cardiac Ca2+ Homeostasis
Ca2+ homeostasis is not as fast in embryonic age as the adult heart; however, it develops to increase its speed regarding Ca2+ transport after the mid-embryonic stage.
Development of SERCA2a activity starts to develop during embryonic stages.
In embryonic and neonatal heart, mitochondrial Ca2+ transport is not as active as adult cardiac cells.
Ca2+ transport through the sarcolemma and the SR of neonatal myocytes is immature; this is mainly due to deficient or incomplete T tubules and also scant and small SR; also, Ca2+ content of the SR is not enough; as far as myocardial tissue development goes on, these structures develop and also Ca2+ content increases to normal levels (Nakanishi et al. 1988; Wetzel et al. 1991; Mahony 1996). In clinical practice, two very important facts are seen:
- 1.
Myocardial contractility in neonates and infants is highly dependent on extracellular Ca2+ stores, since intracellular Ca2+ stores are negligible.
- 2.
Volatile anesthetics suppress myocardial contractility in neonates and children much more than adults, a finding which supports immature SR and limited intracellular Ca2+ stores in this patient population, especially regarding the degree of maturity of NCX; however, some believe that Ca2+ influx channels – not immature SR – are the main mechanism responsible for the effects of volatile agents (Frank et al. 1994; Seckin et al. 2001; Prakash et al. 2002; Park et al. 2007).
- 1.
CICR is not a dominant phenomenon in embryonic heart Ca2+ homeostasis; however, it develops as a cellular mechanism as far as the heart changes from an embryonic heart to a mature type heart.
The compliance of myocardium is less in neonates and infants than older children and adults; in fact, ventricles in neonates and infants are more stiff and do not relax during diastole as much as adult ventricles; this stiffness in part is related to decreased concentration of SERCA2a, RyR, and NCX.
Also, diastolic relaxation in embryonic heart is not as fast as the adult heart; this delay is not only due to decreased speed of Ca2+ kinetics in cytosol but also due to incomplete development of diastole (Kawamura et al. 2010).
L-type Ca2+ channels have a unique model for their development which dominates their role in the heart; so, as age increases, their developmental course changes and improvement in their function happens (Qu et al. 2011).
Developmental Changes in Cardiac Action Potential
Resting membrane potential in neonates is less dependent on K+ current, though this process changes with increasing age which develops to become as in the adult heart (Chen et al. 1991).
Developmental Changes in Mechanical Force Production and Contractile Function
The fetal heart has “low specific force”; as gestation goes on, the force production property of the heart increases and force development is augmented; also, the rate of force development and the rate of relaxation are both slow in the fetal heart; however, this rate increases as much as the embryonic heart develops (Marston and Redwood 2003; Schwan and Campbell 2015; Racca et al. 2016).
The myofibrils of the cardiac muscle elongate and also their width increases as the age of the embryo increases.
M line and Z band are among the very early appearing structures of the cardiomyocyte; they are apparent about day 52 in the fetal period (Schwan and Campbell 2015; Racca et al. 2016).
β-myosin is among those structures and proteins which is seen so much early in cardiac muscle cells (Marston and Redwood 2003).
Cardiac troponin I (TnI) increases progressively while at the same time slow skeletal TnI decreases (Sasse et al. 1993; Schwan and Campbell 2015).
All these changes during gestation of the cardiac myocytes lead to improvements and development of the contractile function; so, these developmental changes are the basis for improved force production as the embryonic heart develops; however, the trend of maturation continues after birth and during childhood and adolescence to lead to the final cardiac structure seen in the adult heart with the contractile properties of the adult heart.Stay updated, free articles. Join our Telegram channel
Full access? Get Clinical Tree
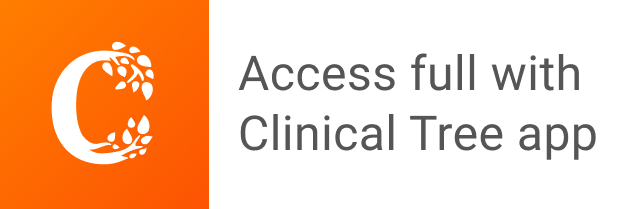