Pathophysiology of Heart Failure: Introduction
Heart failure is a complex clinical syndrome in which the typical symptoms of shortness of breath and fatigue are associated with functional or structural damage to the heart. The diagnosis usually portends an early death preceded by unpleasant symptoms limiting the quality of life. Heart failure is common and becoming more common as the population ages and as patients survive myocardial infarction (MI) with consequent persistent damage to the muscle of the heart. The entity of heart failure is easily recognized by experienced physicians and can be detected in the community, and treatment reduces both morbidity and mortality. Although the molecular biology and integrated physiology of heart failure remain incompletely understood, several concepts and principles have evolved over the past decades. A key feature of heart failure is the impaired ability of the heart to act as a pump. But many body responses, which are secondary adaptive responses maintaining short-term circulatory function, eventually become maladaptive and contribute substantially to the long-term progression of heart failure. These numerous adaptations in response to the onset of heart failure occur in the peripheral circulation, the kidney, skeletal muscle, and almost all organs of the body. The changes contribute to the overall clinical syndrome and phenotype of heart failure. An understanding of how these changes occur provides insight into the pathophysiology of the syndrome and explains why some are therapeutic targets.
Defining Heart Failure and Cardiac Dysfunction
A widely known definition of heart failure is “a pathophysiological state in which an abnormality of cardiac function is responsible for the failure of the heart to pump blood at a rate commensurate with the requirements of the metabolizing tissues.“1 This definition places the emphasis on the physiology of the circulation. More recent definitions have taken a more pragmatic and clinically useful approach. One American definition is “a complex clinical syndrome that can result from any structural or functional cardiac disorder that impairs the ability of the ventricle to fill with or eject blood. The cardinal manifestations of heart failure are dyspnea and fatigue, which may limit exercise tolerance, and fluid retention, which may lead to pulmonary congestion and peripheral edema.“2 A recent European definition is similar: “Heart failure is a complex syndrome that can result from any structural or functional cardiac disorder that impairs the ability of the heart to function as a pump to support a physiological circulation. The syndrome of heart failure is characterized by symptoms such as breathlessness and fatigue, and signs such as fluid retention.“3 The definition of heart failure remains a point of controversy to this day.
Congestive heart failure denotes the clinical syndrome with the features of dyspnea, increased fatigue, and fluid accumulation (jugular venous distension, dependent edema, enlarged liver, and pulmonary edema). Congestive heart failure should be distinguished from cardiac dysfunction, which is a structural definition based on imaging techniques and characterized by abnormal contractility (systolic) or relaxation (diastolic), often accompanied by compensatory ventricular hypertrophy, dilatation (so-called cardiac remodeling), or both. The term cardiomyopathy is often used to describe any condition with evidence of structural abnormalities of the myocardium. The classification of cardiomyopathy can be found in Chap. 33. The nomenclature of heart failure can be confusing because many adjectives are used to emphasize one or another feature (Table 26–1). The extent and progression of ventricular damage (ventricular hypertrophy or dilatation) and the impact on body organs is, in general, related to the neurohumoral/cytokine systemic response. Ventricular performance in general, and when expressed as the left ventricular ejection fraction (LVEF), does not correlate with the ability to exercise. This is because exercise capacity is a composite outcome of ventricular systolic and diastolic function, coupling of the heart and the vasculature, and skeletal muscle conditioning.
Definitions of heart failure |
A pathophysiologic state in which an abnormality of cardiac function is responsible for the failure of the heart to pump blood at a rate commensurate with the requirements of the metabolizing tissues.1 |
Heart failure is a complex clinical syndrome that can result from any structural or functional cardiac disorder that impairs the ability of the ventricle to fill with or eject blood. The cardinal manifestations of heart failure are dyspnea and fatigue, which can limit exercise tolerance, and fluid retention, which can lead to pulmonary congestion and peripheral edema.2 |
Heart failure is a complex syndrome that can result from any structural or functional cardiac disorder that impairs the ability of the heart to function as a pump to support a physiologic circulation. The syndrome of heart failure is characterized by symptoms such as breathlessness and fatigue and signs such as fluid retention.3 |
Congestive heart failure |
Similar to the above definition but with features of circulatory congestion (fluid retention) such as jugular venous distension, rales, peripheral edema, and ascites. Adjectives such as chronic, overt, treated, untreated, undulating, worsening, and compensated can precede the phrase. |
Noncardiac circulatory failure |
A syndrome that is clinically indistinguishable from congestive heart failure in which there is no reason to ascribe the condition to structural heart disease. There must be a noncardiac cause such as acute renal failure. This entity includes so-called high-output heart failure; a better term is circulatory failure because in these conditions, the heart is usually normal. |
Systolic heart failure |
A clinical syndrome with classic symptoms of breathlessness, fatigue, and exercise intolerance whereby the dominant cardiac feature is a large, dilated heart and impaired systolic performance. There may or may not be concomitant valvular disease. |
Diastolic heart failure |
This term is used when the ejection fraction at rest is normal or near normal. An alternative phrase is heart failure with preserved ejection fraction. The features of heart failure are present, and the heart is small or normal in size. There is often left ventricular (LV) hypertrophy and impaired filling of the heart caused by altered LV stiffness or other evidence of diastolic dysfunction. Severe systemic hypertension or valvular disease such as mitral regurgitation can be present. This form of heart failure can coexist with systolic heart failure, particularly with exercise. |
Right-sided heart failure |
A clinical syndrome characterized by tissue congestion, including jugular venous distension, peripheral edema, ascites, and abdominal organ engorgement. There is marked impairment of right ventricular (RV) systolic performance, usually with RV dilatation and severe tricuspid regurgitation. There are multiple causes of this syndrome, including severe left-sided heart failure (the most common cause), severe lung disease with chronic hypoxemia and pulmonary hypertension (cor pulmonale), RV MI, primary pulmonary arterial hypertension, and congenital abnormalities of the heart. |
Left-sided heart failure |
A clinical syndrome in which the dominant feature is fluid congestion in the lung (pulmonary edema) rather than in the systemic circulation. |
Acute heart failure |
Some physicians limit this term to a serious medical emergency synonymous with acute pulmonary edema. In recent years, the meaning of the phrase has been extended to include new-onset heart failure and worsening heart failure on a background of chronic heart failure. |
The spectrum of biologic processes involved depends on the nature and temporal sequence of alterations leading to the clinical presentation. The acute heart failure syndrome (or acute decompensated heart failure or simply acute heart failure commonly characterized by pulmonary edema) is defined as a short-term or rapid change in heart failure signs and symptoms resulting in a need for urgent therapy.4 These symptoms can develop in a progressive manner over a short period of time (hours, days, or weeks), sometimes with a defining event such as during acute regional ischemia or necrosis of the ventricle (eg, accute MI), recent onset of atrial fibrillation, other arrhythmias, or sudden loss of valve function such as that caused by rupture of a papillary muscle or chordae tendinea. An acute shift of blood volume from the systemic to the pulmonary circulation (sometimes referred to as flash pulmonary edema) can even occur before significant salt or water retention has ensued. Acute heart failure is distinguished from chronic heart failure, which refers to a relatively more stable but symptomatic condition, in many cases being considered as compensated heart failure. Specific factors involved in converting from a compensated to a decompensated state in any individual patient with heart failure can vary, are not well understood, and can take place over days to weeks. In chronic heart failure, fatigue can occur because of a limited cardiac output and neurologic signals from underperfused and damaged skeletal muscle. Fluid accumulation can occur, leading to pulmonary congestion and peripheral edema, that is, congestive heart failure.
A more contemporary distinction in patients with heart failure is to characterize the particular structural abnormalities with cardiac imaging techniques, and the majority of clinical studies in heart failure have used this phenotyping. Systolic dysfunction describes a large, dilated, and often eccentrically hypertrophied ventricle in which output is limited by impaired ejection during systole. Diastolic dysfunction refers to a thickened, small cavity ventricle in which filling is limited because of abnormalities during diastole (Table 26–2). These terms are most appropriately defined in terms of altered ventricular performance and geometry rather than systemic hemodynamics or overt symptoms because they can manifest themselves with almost identical symptomatology. It is also clear that systolic and diastolic dysfunction frequently coexist in patients with heart failure because systolic dysfunction, notably with exercise, can directly influence diastolic function. Systemic symptoms may not correlate with the degree of ventricular dysfunction as assessed by contraction during systole at rest.
Systolic Heart Failure | Diastolic Heart Failure |
---|---|
Large, dilated heart | Small LV cavity, concentric LV hypertrophy |
Normal or low blood pressure | Systemic hypertension |
Broad age group; more common in men | Elderly women more common |
Low ejection fraction | Normal or increased ejection fraction |
S3 gallop | S4 gallop |
Systolic and diastolic impairment by echo | Diastolic impairment by various echo measurements |
Treatment well established | Treatment not well established |
Poor prognosis | Prognosis is poor after hospitalization is required for heart failure |
Role of myocardial ischemia important in selected cases | Myocardial ischemia common |
Because both sides of the heart are part of a circuit in series, one side of the circulation cannot pump significantly more blood than the other for any length of time in the absence of abnormal shunts, communications, or regurgitation. In most situations, the expression left heart failure (or pump failure) is used clinically in reference to symptoms and signs of elevated pressure and congestion in the pulmonary veins and capillaries, and the term right heart failure refers to symptoms and signs of elevated pressure and congestion in the systemic veins and capillaries characterized by jugular vein engorgement and hepatic congestion. Abnormal function of the left ventricle (LV) with impaired forward flow not only overloads the right ventricle from augmented pulmonary pressures (the old-fashioned term was backward heart failure) but can also affect the right ventricle by means of the shared septum. Therefore, right-sided heart failure commonly occurs after left-sided heart failure. However, significant amounts of sodium and water retention, with subsequent peripheral edema formation, can occur with pure left-sided heart failure without hemodynamic evidence of right-sided heart failure. This occurs because reduced perfusion of the kidney leads to salt and water retention (old-fashioned forward failure). An increase in the diastolic volume in either ventricle can increase the diastolic pressure or decrease the distensibility of the contralateral ventricle, especially if the pericardium is intact; the biochemistry and hemodynamics of the contralateral ventricle can also be abnormal even in “pure“ one-sided failure.
Pathophysiologic Concepts of Heart Failure
The causes of heart failure are classified into six main categories: (1) failure related to an abnormality of the myocardium, which can be caused by loss of myocytes (eg, MI), incoordinate contraction (eg, left bundle-branch block, right ventricular pacing), reduced contractile force (eg, cardiomyopathy or cardiotoxicity) or disorientation of cells (eg, hypertrophic cardiomyopathy); (2) failure primarily related to external work overload (eg, hypertension or aortic stenosis); (3) failure related to valve abnormalities; (4) failure caused by an abnormal cardiac rhythm (eg, incessant tachycardia); (5) failure caused by pericardial abnormalities or a pericardial effusion (tamponade); and (6) congenital deformities of the heart. Because any form of heart disease can lead to heart failure, there is no single causative mechanism. Likewise, at the organ and the cellular levels, there is no single mechanism that accounts for malfunction of heart muscle, although identification of fundamental mechanisms remains an area of very active investigation. Multiple subsequent alterations in organ and cellular physiology contribute to the syndrome of heart failure under various circumstances and at different points in time (Table 26–3). For example, with myocardial failure, ventricular dilatation can occur, maintaining stroke volume (SV; the Starling effect). This dilatation can lead to failure of the mitral valves to close completely, resulting in mitral regurgitation. This then creates a secondary functional volume load for the already compromised LV. Adaptive processes in the periphery occur that can adversely affect the myocardium, kidneys, smooth and skeletal muscles, endothelium, peripheral vasculature, and multiple reflex control mechanisms, adding to the complexity of the syndrome (Table 26–4). The schema of the sequence of events in heart failure is daunting. Distinguishing primary etiologic forces from secondary epiphenomena is difficult. Identification of the precise mechanisms whereby heart failure evolves and quantifying the contributions of individual components such as a primary decrease in capacity of myocytes to shorten adequately (eg, decreased contractility) or loss of myocytes (eg, apoptosis or myocardial necrosis) has remained elusive. Indeed, loss of myocytes with compensatory dilatation of the ventricle can lead to further loss of myocytes. What triggers the early activation of the sympathetic nervous system and withdrawal of vagal tone and how spontaneous resolution of heart failure occurs remain unclear. Nevertheless, enough information has accrued to construct a reasonably coherent working hypothesis.
Loss of myocytes |
Hypertrophy of remaining myocytes |
Energy production and utilization |
Oxygen and energy supply |
Substrate utilization and energy storage |
Inadequate mitochondria mass and function |
Ventricular remodeling |
Contractile proteins |
Abnormal myofibrillar or myosin ATPase |
Abnormal myocardial proteins |
Defective protein synthesis |
Nonuniformity of contraction and function |
Activation of contractile elements |
Membrane Na+,K+-ATPase defects |
Abnormal sarcoplasmic reticulum function |
Abnormal Ca2+ release |
Abnormal Ca2+ uptake |
Abnormal myocardial receptor function |
Downregulation of β adrenoreceptors |
Decreased β1 receptors |
Decreased Gs protein |
Increased G1 protein |
Autonomic nervous system |
Abnormal myocardial norepinephrine function or kinetics |
Abnormal baroreceptor function |
Increased myocardial fibroblast growth and collagen synthesis |
Aging changes, presbycardia |
Sustained tachycardia |
Miscellaneous |
Autonomic nervous system |
Heart |
Increased heart rate |
Increased myocardial contractile stimulation |
Increased rate of relaxation |
Peripheral circulation |
Arterial vasoconstriction (increased afterload) |
Venous vasoconstriction (increased preload) |
Kidney (renin–angiotensin–aldosterone system) |
Arterial vasoconstriction (increased afterload) |
Venous vasoconstriction (increased preload) |
Sodium and water retention (increased preload and afterload) |
Increased myocardial contractile stimulation |
Endothelin-1 (increased preload and afterload) |
Arginine vasopressin (increased preload and afterload) |
Atrial and brain natriuretic peptides (decreased afterload) |
Prostaglandins |
Peptides |
Frank-Starling law of the heart |
Increased end-diastolic fiber length, volume, and pressure (increased preload) |
Hypertrophy |
Stem cell maturation replacing lost myocardium |
Peripheral oxygen delivery |
Redistribution of cardiac output |
Altered oxygen-hemoglobin dissociation |
Increased oxygen extraction by tissues |
Anaerobic metabolism |
The growth of genetics and molecular cardiology has provided important insights into the pathophysiology of heart failure (see Chaps. 6, 7, 8, and 11). However, because the cause and progression of heart failure is complex, both environment and genetics play important roles. Thus, there is no single cause or unifying mechanism of heart failure, and current therapeutic strategies target multiple pathophysiologic processes.
Alterations are found in the failing heart in numerous contractile proteins, especially in heredity-based idiopathic dilated cardiomyopathies. In the latter situation, these alterations can interact with abnormal loading conditions to cause heart failure. Such alterations have been found in the proteins of the cytoskeleton, myosin, troponin T, and actin and are likely to contribute to diminished myocardial performance. In animal models, various overloads can result in heart failure, whether from systolic loads (hypertension), loss of myocardium (infarction), inflammation, and so forth. Furthermore, in human failing hearts, the etiology can be modified from that in animal models. In the failing human heart, many changes in gene expression at the mRNA or protein level have been found in failing hearts harvested at the time of cardiac transplantation. However, these are often hearts with end-stage myocardial disease in which many factors (eg, receiving multiple inotropic drugs) can obscure the initial pathogenesis.
More than half of the volume of cardiac myocytes contains contractile proteins. Myosin comprises the thick filament that hydrolyzes adenosine triphosphate (ATP), interacts with the thin actin filaments to produce force and shortening. Two myosin heavy-chain (MHC) isoforms are present in mammalian heart, α− and β−MHC. The α−MHC is cardiac specific and is more enzymatically active. The less active β−MHC is present in the heart and in slow-twitch skeletal muscle. The distribution of α− and β−MHC is developmentally and hormonally regulated. Mechanical stress, such as pressure overload, induces an α− to β−MHC transition in the ventricles of experimental animals, thus imparting a slower but more economical type of work for the overloaded heart. Either way, myosin remains a principal structural and contractile unit of muscle fiber.
There is a general agreement that myofibrillar function is depressed in the failing human heart, but its causal role remains controversial. Downregulation of α−MHC and upregulation of β−MHC using mRNA measurements from right ventricular endomyocardial biopsies from nonfailing hearts and failing human hearts has been demonstrated,5 and their sequential changes can be observed with recovery of cardiac performance.6 This alteration, if translated into protein expression, would decrease myosin adenosine triphosphatase (ATPase) enzyme velocity and slow the speed of contraction. Although such adaptive changes could be viewed to have an economical survival advantage in the face of increased load, slower contraction and relaxation could also contribute to diastolic dysfunction. In addition, isoform changes involving both the heavy and the light chains, as been suggested, can play a role in heart failure. In contrast to smaller mammalian species such as mouse, rat, and rabbit, the normal human ventricle contains at least 90% slow β−MHC, so an isoform shift to increase the large amount of β−MHC already in the cell is quantitatively less important than in small mammals, and the functional significance is unclear.
In addition to myosin heavy- and light-chain isoform switches, other well-understood pathologic events also contribute to altered cardiac function in various forms of heart failure. Point mutations of virtually all of the sarcomeric proteins cause hypertrophic cardiomyopathy (see Chap. 35). Similarly, mutations in the cytoskeletal proteins that provide the molecular scaffold for the sarcomere have been found in both dilated and hypertrophic cardiomyopathies. Echocardiographic studies have demonstrated that 20% of first-degree relatives of patients with idiopathic dilated cardiomyopathy have enlarged LV cavities (see Chap. 34)
Plasma membrane ion channels initiate excitation–contraction by generating and then propagating the action potentials that depolarize the myocardium. These ion channels are complex and contain several subunits that surround the ion-selective pore. The intracellular calcium (Ca2+) release channels are found in the sarcoplasmic reticulum (SR) and are quite different from those of the plasma membrane. The SR Ca2+ release channels are referred to as ryanodine receptors (RyRs) and interact with the ligand inositol triphosphate (IP3). The Ca2+ pump ATPases are found in both the plasma membrane and SR. Both calcium pumps are activated by cytosolic Ca2+. The sodium-calcium exchanger (NCX) transports calcium out of the cytosol into the extracellular space, using the osmotic energy of the sodium gradient across the plasma membrane to generate active transport. The sodium-potassium (Na+,K+)-ATPase pump uses energy derived from ATP hydrolysis to exchange sodium that enters the cell for potassium lost from the cytosol during repolarization. Also, calcium-binding storage proteins (eg, calsequestrin) maintain a calcium store that can be readily used during excitation–contraction coupling. The heart’s voltage-gated ion channels (especially inward sodium channels) are altered in the failing heart,7 as are outwardly rectifying potassium channels (see Chaps. 8 and 38).8 A common feature of animal models of heart failure and clinical heart failure in humans is prolongation of the action potential. Both decreased sodium influx and potassium efflux are contributory and are mediated by reduced activity of the sarcolemmal sodium and potassium channels, respectively. This aberrant channel behavior contributes to the arrhythmias, which are the second most common cause of death of patients with heart failure. Multiple additional mechanisms are also contributory, including excess sympathetic stimulation and extracellular matrix–induced scar formation.
Excitation–contraction coupling links plasma membrane depolarization to the release of calcium into the cytosol, where it binds to troponin C, permitting the force-generating interaction between myosin and actin. Relaxation is also an energy-dependent process but is not simply a reversal of the steps in excitation–contraction coupling. During relaxation, calcium is actively transported out of the cytosol by entirely different structures. The basic mechanism of cardiac excitation–contraction coupling involves calcium (Ca2+) entry from the extracellular fluid by means of the voltage-dependent L-type calcium channel to produce a trigger in increasing [Ca2+]i and opening of the intracellular SR Ca2+ release channel or RyR. Defects in sarcolemma Ca2+ uptake (sarcolemmal transport via Na+,K+-ATPase) and release by the SR are present in heart failure, especially at later stages,9 but uptake of calcium by the SR can remain intact. These alterations in calcium transport can be secondary to quantitative alterations of gene expression of SR calcium transport proteins, especially the sarcoplasmic–endoplasmic reticulum calcium ATPase (SERCA) and phospholamban, a reversible inhibitor of cardiac SR Ca2+-ATPase activity. Other calcium-cycling proteins such as Na+-Ca2+ exchanger proteins10 can also be altered in heart failure. Phosphokinase A hyperphosphorylation of RyR has recently been shown to alter calcium signaling from the SR by depleting calcium stores and reducing calcium transients that can impair contractility in the failing myocardium.11 Several of these alterations can occur concurrently and can vary from model to model and may not always be relative to failing human hearts. Nevertheless, it is likely that heart failure is characterized by reduced myofilament activation and decreased calcium available for activation as well as heightened cytosolic calcium levels in diastole. Some studies have shown increased myofibrillar calcium sensitivity12 and altered calcium kinetics. These abnormalities of calcium metabolism can be of primary importance in some types of heart failure, and they can be secondary or epiphenomena in other types. Most abnormalities of myocardial contractile activation have been demonstrated only in the late stages of heart failure and therefore can be the result of maladaptive hypertrophy rather than a primary cause of ventricular dysfunction. Molecular and cellular mechanisms for pathologic cardiac hypertrophy and failure are reviewed in detail in Chap. 8.
High energy phosphate levels are reduced in both animal models of heart failure13 and failing human hearts.14 Levels of phosphocreatine (PC) are more depressed than ATP. Reduced PC levels impair the shuttle that normally transfers energy from the mitochondria to the cytosol. The abnormal pattern of energy production in the failing heart resembles that of the fetal heart. This is also true of abnormalities in excitation–contraction coupling, myocyte contraction, and myocyte relaxation. In essence, the failing (and fetal) heart is less reliant on the more efficient pathways of mitochondrial ATP production. Less high-energy phosphates are available to meet the increased work demands of the failing heart. Even a small reduction in the phosphorylation potential impairs ATP-dependent reactions because the heart has only a small phosphorylation reserve capacity. Moreover, decreased PC levels reflect more PC use rather than a lack of adequate PC.
Oxygen deprivation, which is most often caused by coronary artery disease, results in impaired relaxation and weakened contraction, as can be seen in transient angina pectoris. This is readily reversible. With prolonged ischemia, decreased contraction (dyskinesis) can persist for hours beyond return of blood flow (stunning). If coronary blood flow is chronically reduced, the myocardium can fail to contract normally (hibernation), even if necrosis does not ensue (see Chap. 54). With a more serious loss of flow, infarction can occur. All of these stages can produce substantial dyskinesia for which the remaining myocardium must sustain this load. The result is hypertrophy of the nonischemic portion of the ventricle; if this is inadequate, an increase in ventricular volume occurs using the Frank-Starling mechanism to sustain SV. Whether there is a true limitation of energy supply or its use in the failing myocardium remains controversial.
In patients with heart failure, the total oxygen requirement of the heart can be increased significantly because of the increased total mass, the increase in myocardial systolic wall tension because of the Laplace relationship, and perhaps some wasted contractile energy. This increase can result in the extraction of a greater amount of oxygen from each unit of coronary blood flow and a widening of the coronary arteriovenous oxygen difference. Many patients with heart failure are able to increase coronary blood flow during exercise; however, some patients with a dilated ventricle that increases in diameter during exercise can have a further widening of the coronary arteriovenous oxygen difference during exercise and a decrease in coronary blood flow reserve (see Chaps. 4 and 40). In the presence of severe LV hypertrophy, coronary blood flow per unit mass of myocardium is usually normal at rest. Conversely, the capacity of the coronary vascular bed to dilate during reactive hyperemia, which is normally four- to five-fold, is reduced in the presence of severe hypertrophy when filling pressures are elevated. Tachycardia, such as can occur with atrial fibrillation, can reduce diastolic time for coronary perfusion, producing ischemic ventricular failure. Although reduced perfusion is probably common in end-stage heart failure, a deficit in coronary blood flow or oxygen delivery has not been clearly demonstrated to be a primary cause of heart failure associated with hypertrophy except in the presence of obstructive coronary disease (see below).
Although the myocardial uptake of fatty acids and glucose per 100 g of myocardium is normal in heart failure, there is conflicting evidence on whether or not there is a primary decrease in energy liberation by mitochondrial oxidative phosphorylation. The reductions in stores of myocardial high-energy phosphate, creatine phosphate, or ATP generally found in heart failure are thought to be secondary. This can be a consequence of the failure rather than the primary cause of the failure. There can also be reduced levels of creatine kinase and changes in the isoenzymes of creatine kinase in heart failure.
The major consequences of the state of energy starvation that is observed in failing hearts are caused by attenuation of important allosteric (regulatory) effects of ATP rather than a reduction in the supply of substrate for the many energy-consuming reactions involved in contraction, relaxation, and excitation–contraction coupling. By facilitating the many calcium fluxes involved in excitation–contraction coupling and relaxation, these allosteric or regulatory effects of ATP exert both inotropic and lusitropic effects (see Chap. 8).
There are conflicting data on whether there is a significant decrease in the mass of mitochondria relative to the mass of myofibrils that occurs in experimental cardiac hypertrophy. It is possible that this is one of the limitations of severe hypertrophy. Defects in mitochondrial oxidative phosphorylation and in mitochondrial calcium metabolism can also be associated with myocardial failure. Except when coronary flow is limited, such as with large vessel obstructive disease (see Chap. 43) or purported microvascular obstructive or vasospastic disease, a primary role of energy limitation in the evolution of heart failure has yet to be demonstrated. It is possible that it plays a role during periods of higher metabolic demand, such as tachycardia, as noted previously.15 Furthermore, mitochondrial dysfunction can produce increased reactive oxygen species (ROS) that can produce additional damage and death of myocytes.
Systolic ventricular dysfunction as a result of focal loss of contraction can be dynamic and transient as can occur with acute ischemia. With restoration of metabolic requirements of an ischemic segment of myocardium, either from restoring adequate coronary flow or reducing oxygen requirements, myocardial contraction can be restored. Sometimes restoration is delayed, so-called stunning. Chronically reduced coronary flow can be inadequate to preserve contraction but adequate for myocardial survival. Such persistent depressed myocardium has been termed hibernation and with reperfusion can recover contractility over a period of time (see Chap. 53).
Heart failure is characterized by many abnormal reflex control mechanisms. Peripheral vascular resistance is increased, and there is defective cardiac parasympathetic control, an abnormal response to upright tilt, altered baroreceptor function,16,17 and reduced cardiac sympathetic activity in response to a variety of stimuli.18-20 Indeed, an early sign of heart failure is increased sympathetic tone accompanied by reduced vagal tone, resulting in a modest increased heart rate even at rest.
An increase in systemic vascular resistance (SVR) is generally observed in well-established heart failure. It is likely caused by a combination of locally active heightened vasoconstrictors (norepinephrine [NE], angiotensin II, endothelin, vasopressin, neuropeptide Y) and by structural changes in blood vessels from fluid retention and reduced endothelial-dependent vasodilatation. These later changes are closely associated with limitations of exercise in heart failure. Early in heart failure, there may be a decrease in cardiac output, arterial pressure, and baroreceptor activity, leading to an adaptive increase in excessive neuroendocrine drive. The sympathetic nervous system is activated early, followed by the renin–angiotensin–aldosterone system (RAAS). Arginine vasopressin (AVP) is released. Sodium and water retention occur, hypervolemia restores cardiac output and arterial pressure, and neuroendocrine activity can reach a steady state. However, as heart failure progresses, there is impaired cardiosensory activity that fails to reduce neuroendocrine drive. For unclear reasons, cardiac afferent activity to the central nervous system is reduced, leading to unhindered, efferent excitatory responses from the brain to the periphery. Reflex vasoconstrictor responses to unloading the heart are paradoxically blunted.20 There are abnormal vascular responses to postural change. Some of these changes lead to alterations in regional blood flow that accompany heart failure. Parasympathetic (vagal) tone is decreased, and heart rate variability is markedly reduced, a hallmark of congestive failure. Furthermore, decreased heart rate variability can provide independent prognostic value in the identification of patients at risk for premature death.21
Although the genesis of these abnormal reflex control mechanisms is poorly understood, the changes can be more functional than structural. Heart transplantation reverses cardiopulmonary baroreflex control mechanisms to some extent, but this is inconsistent. The role played by abnormal reflex control mechanisms in the progression of heart failure, similar to other neuroendocrine alterations, has been difficult to quantify. Nevertheless, it is now increasingly clear that the sympathetic nervous system and the RAAS greatly influence the progression and natural history of heart failure with very important therapeutic implications.
The failing heart commonly demonstrates a decreased response to inotropic stimuli. Although no single mechanism accounts for this, the reduction in myocardial β−adrenergic receptors and the subsequent second messenger cyclic adenosine monophosphate (cAMP) can play an important role.22 β−Adrenergic stimulation contributes importantly to the cardiac response to exercise, and β−adrenergic desensitization and uncoupling can be at least partially responsible for the reduced chronotropic and inotropic response to peak exercise commonly found in patients with heart failure. The β−adrenergic receptor abnormalities in heart failure appear to be caused by desensitization and uncoupling of the β1 receptor produced by local rather than systemic alterations in catecholamines. In severe heart failure, the NE stores in sympathetic nerve endings are depleted. In a sense, the failing myocardium becomes functionally denervated. cAMP responses are reduced by approximately 30% to 35%, leading to further contractile dysfunction. Despite downregulation of the β1 receptor, a relatively high proportion of β2 receptors remains to mediate chronotropic and inotropic responses.23 However, there is some uncoupling of the β2 receptor from its G protein and a modest upregulation of the Gαi subunit, further contributing to a depressed response to chronotropic and inotropic stimuli.24 There is also a profound decrease in cardiac β−adrenergic responsiveness with aging,25 which has clinical implications because heart failure is heavily concentrated in the elderly population.
The desensitization and uncoupling of β−adrenergic receptors that occurs early with mild to moderate ventricular dysfunction is related to the degree of heart failure and is associated with a very reduced response to β−adrenergic stimulation with drugs such as dobutamine. Long-term stimulation of β−adrenergic receptors can enhance myocardial β−adrenergic receptor kinase (β−ARK) activity,26 leading to further desensitization and uncoupling of the β−adrenergic receptor.
Of great therapeutic interest, β−adrenergic blockade with metoprolol, a relatively cardioselective β1-blocker, upregulates the β1 receptor, but carvedilol, a nonselective β1- and β2-blocker with additional α1 blocking activity, does not increase β1 receptor density.27 Both drugs improve LV function substantially in approximately two-thirds of patients. The ventricular improvement seen with chronic β-blocker use may not be caused by upregulation of β−adrenergic receptors, and the beneficial effects of β−adrenergic receptor blockade in heart failure remains unexplained. Moreover, high plasma NE levels do not predict benefit from carvedilol,28 suggesting that there is not a simple relationship between activation of the sympathetic nervous system and response to β−adrenergic blocking drugs in patients with heart failure.
The failing human myocardium is characterized by an abnormal force–frequency response that parallels the severity of heart failure. Normally, an increase in the frequency of stimulation is accompanied by an increased rate of force development, a decreased duration of contraction, and an enhanced rate of relaxation (Bowditch effect). This tends to preserve or increase contractile force while preserving diastolic time. The latter effect is important in the tachycardic intact heart in preserving time in diastole to prevent ventricular filling and coronary blood flow. In isolated failing heart muscle, an increase in heart rate has been accompanied by a decrease in myocardial performance. Some impairment of systolic function in response to increased heart rate can also be related to impaired LV filling, although a negative inotropic effect as shown in isolated muscle has been related to alterations in intracellular Ca2+ handling. A reduced force of contraction and lack of shortening of contractile activity can contribute importantly to impairment of cardiac function during exercise.29,30
The term heart failure implies structural heart disease, and the central problem of heart failure remains impaired cardiac performance, although many of the secondary adaptive responses become maladaptive and contribute substantially to the progression of heart failure. An understanding of how these changes occur can provide insight into the pathophysiology over the course of the syndrome (see Chap. 6).
In patients with mild heart failure, the ventricular end-diastolic pressure (EDP) and the cardiac output can be normal at rest, but the former can become elevated to abnormal levels during stress such as exercise with increased cardiac output or an increase in afterload as the blood pressure increases. The ability to increase the cardiac output in response to the increase in oxygen consumption is also reduced (see below and Chap. 4). In patients with more severe systolic dysfunction, the EDP can be elevated even at rest. As the diastolic volume is increased, so is the end-systolic volume. This results in reduced elastic recoil of the ventricle during relaxation and is reflected in loss of rapid early diastolic ventricular filling (as revealed by a reduced E wave of the echocardiogram). This helps further increase the mean diastolic pressure. The elevated LV diastolic pressure increases pulmonary venous and capillary pressures and contributes to increased dyspnea as a result of changes in pulmonary compliance because of pulmonary congestion and edema. Before one reaches this stage of clinical heart failure, the body has used many compensatory mechanisms, but compensatory mechanisms eventually have failed.
Myocardial failure can develop from many causes of overload. In pressure overload, myocytes hypertrophy to overcome the increased demands of the excess load. Hypertrophied cells contract and relax more slowly and can be subject to metabolic limitations. In addition, hypertrophied myocardial cells can have a shortened lifespan. This is of considerable prognostic importance because cardiac myocytes appear to have a reduced capacity to proliferate. When age-related myocyte loss is added to the picture, particularly in association with a late decrease in myocyte contractile activity, diastolic failure can ensue. As the process continues, ventricular dilatation can occur with systolic failure as well. Loss of myocytes—whether segmental, as in acute MI, or diffuse, as in myocarditis—sets up a vicious cycle that leads to reactive hypertrophy in the remaining myocytes. As compensatory hypertrophy becomes more marked in some disease states, the contractility unit of the myocardium often declines because of molecular changes in the heart’s contractile proteins and activation system. This is especially likely to occur in response to pressure overload, as in systemic arterial hypertension or aortic stenosis, but also ensues when myocytes are lost from any mechanism.
As ventricular function becomes impaired, the Frank-Starling law of the heart becomes operative (Fig. 26–1). Inadequate emptying of the ventricle leads to increased end-diastolic volumes (EDVs). This is referred to as increased preload, and it produces an increase in SV during the next contraction. The Frank-Starling law simply states that the increase in contractile force (ie, contractility) is related to sarcomere lengthening (≤2.2 μm). For any given amount of Ca2+ released into the myocyte, there is increased crossbridge formation and enhanced sensitivity of the myofilament to Ca2+ as the sarcomeres lengthen.
FIGURE 26–1.
Relationship between stroke volume (SV) and left ventricular (LV) end-diastolic pressure (LVEDP) (left) and afterload (right). Normally, the ventricle operates on a sharply rising Frank-Starling curve with an LVEDP less than 12 mm Hg (point A), where small changes in filling pressure yield large changes in SV. Furthermore, SV is largely independent of the afterload. When failure occurs, ventricular function is characterized by a shift of the curve relating SV to LVEDP to the right and downward. Whereas low output can ensue if the curve is sufficiently depressed, pulmonary congestion occurs as the LVEDP is increased. At the same time, this failing ventricle is now highly afterload dependent in that small changes in afterload produce large changes in SV. When afterload is reduced in the normal heart (point A to point B, right), SV rises very slightly. If, at the same time, venodilation reduces filling pressure, SV falls to point C (left). The net result is a decrease in cardiac output. On the contrary, when afterload is reduced in the presence of severe ventricular failure, SV is increased (point D to point E, right). Because the Frank-Starling curve is relatively flattened, a simultaneous decrease in filling pressure leads to a decrease in LVEDP with only a small decrease in SV (point E to point F, left). The net result of these opposing consequences can be an increase in SV . These results are observed clinically when a vasodilator is administered together with a diuretic in treating the failing ventricle.
In the failing ventricle, the extent of shortening for a given diastolic fiber length and load (afterload) is reduced. The ventricle can maintain a normal or near-normal SV with an increased EDV and thus maintain end-diastolic fiber length for a period of time. Eventually, the filling pressure increases inordinately, limiting this compensation. Furthermore, the clinically dilated ventricle tends to relax like an overstretched elastic band, and EDV can increase somewhat with no increase in LV EDP, reflecting a shift in the passive pressure–volume curve to the right. An obligatory reduction in EF occurs when SV is maintained in the face of a large EDV (EF = SV/EDV; normal EF = 0.62 + 0.12). Eventually, further increases in EDP produce little change in EDV, thus flattening the SV–EDP curve (see Fig. 26–1). There is no true descending limb to the Frank-Starling curve because increasing preload indefinitely will ultimately lead to mitral regurgitation, displacing the increased load created by the Laplace relation. As the heart dilates, the increase in wall stress according to the Laplace relationship will also increase afterload, which can account for any observed reduction in SV as the heart dilates further (ie, the perception of a descending limb) (see Fig. 26–1). It is important to keep in mind that LV performance depends not only on systolic pump function but also on active relaxation, diastolic elastic recoil, passive diastolic properties, and vascular loading conditions. It is likely that at high LV EDP, valvular incompetence (mitral regurgitation) is a major cause of a decrease in cardiac output. Thus, in end-stage heart failure in the intact circulation, the Frank-Starling curve flattens out. It is possible under certain experimental conditions that the severely failing heart is able to use the Frank-Starling mechanism, but a hallmark of heart failure is the inability of the chamber to respond robustly to an increase in preload.
A characteristic feature of the dilated, failing heart is that it gradually becomes less sensitive to preload (EDV and fiber length) and more sensitive to afterload stress. At very high LV filling pressures (>30 mm Hg) when the sarcomeres are fully extended and the preload reserve is exhausted, the SV becomes exquisitely sensitive to alterations in the afterload. The impedance to ejection includes blood viscosity, vascular resistance, vascular distensibility, and myocardial wall tension. The afterload is the total load that the heart must work against during contraction. Much of the afterload is made up of ventricular myocardial wall tension. In the ventricle, the tension on the walls increases as ventricular chamber volume increases even if intraventricular pressure remains constant. As the ventricle empties, tension is reduced, even as pressure increases. Calculations of myocardial wall tension are defined by the Laplace equation and are expressed in terms of tension, T, per unit of cross-sectional area (dynes per centimeter [dyn/cm]).
Within a cylinder, the law of Laplace states that wall tension is equal to the pressure within the cylinder times the radius of curvature of the wall:
where T is wall tension (dyn/cm), P is pressure (dyn/cm2), and R is the radius (cm). Basically, wall tension is proportional to the radius. Because the heart has thick ventricular walls, wall tension is distributed over a large number of muscle fibers, thereby reducing tension on each. The equation for a thick-walled cylinder such as the heart is:
where h is wall thickness. The equation is sometimes stated as:
Because the geometry of the ventricles is more complex than that of a cylinder, ventricular wall tension cannot be measured with precision. Wall stress, the force distributed across an area, is actually more correct but is seldom measured.
Two fundamental principles stem from the relationship between the geometry of the ventricular cavity and the tension on its muscular walls:
Dilatation of the ventricles leads directly to an increase in tension on each muscle fiber.
An increase in wall thickness reduces the tension on any individual muscle fiber. Therefore, ventricular hypertrophy reduces afterload by distributing tension among more muscle fibers.
The wall tension is highest in the inner surface of the heart. The endocardial surfaces must do more work and therefore are also more vulnerable to reductions in coronary blood flow. Dilatation of the heart decreases cardiac efficiency unless hypertrophy is sufficient to normalize wall stress. In heart failure, wall tension (or stress) is high, and thus afterload is increased. The energetic consequences of the law of Laplace can have some role in progressive deterioration of energy-starved cardiac myocytes in the failing heart.
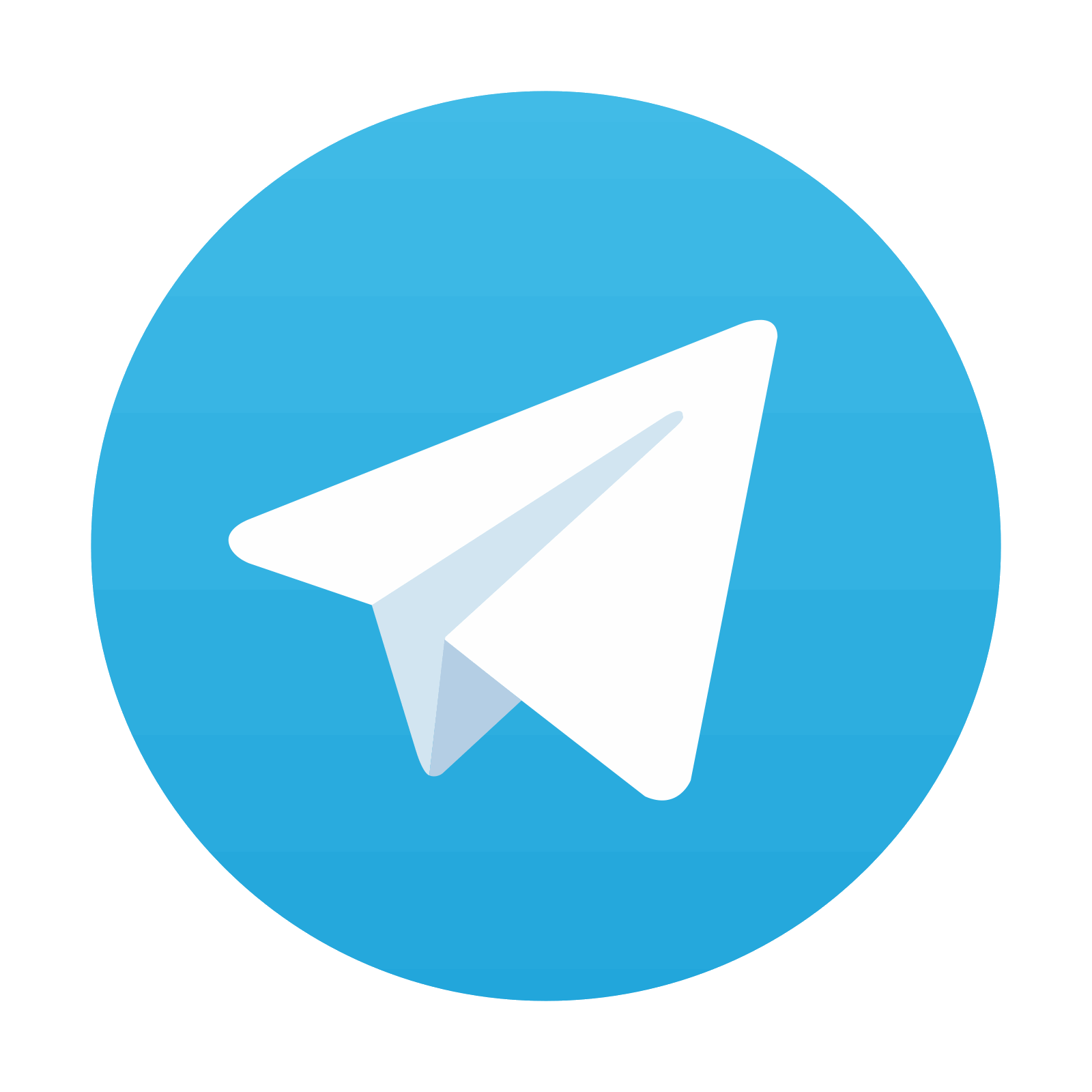
Stay updated, free articles. Join our Telegram channel
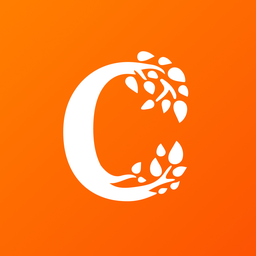
Full access? Get Clinical Tree
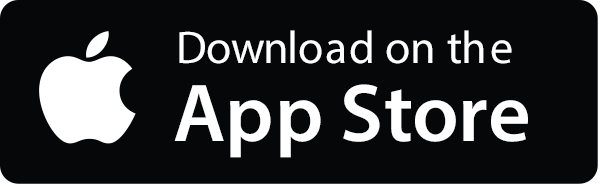
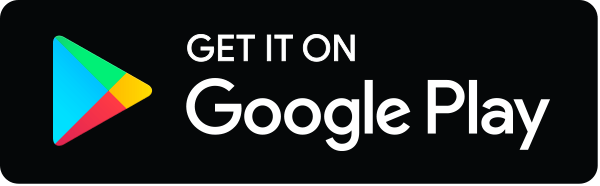