Fig. 7.1
Spectrum of sleep apnea (SA) syndromes along the obstruction vs. chemoreflex axis. 1 idiopathic central sleep apnea (ICSA). 2 high-altitude SA. 3 congestive heart failure with nearly pure Cheyne-Stokes breathing. 4 NREM-dominant obstructive SA. 5 hypoventilation syndromes. 6 REM-dominant SA. There is an intrinsic dynamism in this group. Examples: CPAP can shift towards central apnea (green arrow, may reach threshold for currently defined complex SA); acetazolamide, O2 or CO2 can shift to a more pure obstructive phenotype and thus CPAP responsiveness (red arrow); the worst outcome could be both worsening obstruction and heightened chemosensitivity (blue arrows), such as weight gain + congestive heart failure (the latter group can demonstrate the whole range of obstruction and chemosensitivity)
Sleep and Respiratory Control
Non-rapid eye movement (NREM) sleep unmasks a highly sensitive hypocapnia-induced apneic threshold, whereby apnea is initiated by small transient reductions in arterial CO2 partial pressure (PaCO2) below eupnoea, and respiratory rhythm is not restored until PaCO2 has risen significantly above eupneic levels [2–10]. The CO2 reserve (the difference in PaCO2 between eupnoea and the apneic threshold, determined by plant gain (the change in blood gasses in relation to change in ventilation) and controller gain (the change in ventilation/respiratory driuve in relation to change in O2/CO2; the ventilatory responsiveness to CO2 above eupnoea) is a key determinant of breathing instability in sleep. The CO2 reserve varies inversely with both plant gain and the slope of the ventilatory response to reduced CO2 below eupnoea. The reserve is highly labile in NREM sleep [4]. Reductions in cerebrovascular responsiveness to CO2 result in a gain in chemoreflex control of sleep-breathing, and may also play an important role in mediating respiratory instability during sleep [11–13]. Both central (especially the ventral medullary surface) and peripheral (especially carotid body) chemoreceptors are known to mediate chemoreflex control of respiration [7, 14–17]. During the wake state, top-down influences including from the cortex are important determinants of ventilation. During sleep, the system largely functions automatically during NREM sleep, while REM sleep seems to have unique respiration characteristics. Studies that have tried to dissociate the central and peripheral components seem to suggest that rapid responses are dependent on the peripheral chemoreceptors [7, 9, 18].
Respiratory Chemoreflex Enhancement and Disease
A primary increase in the slope of the respiratory chemoreflexes is best described with the hypercapnic ventilatory response. The quintessential disorder is “idiopathic central sleep apnea” (ICSA)—a rare sleep disorder, where the single consistent abnormality is an increased slope of the hypercapnic response [19–22]. An increase that is at least partially reversible in hypoxic and hypercapnic ventilatory responses occurs in obstructive sleep apnea syndromes [23]. Hypoxia, heart failure, or increased pulmonary vascular pressures all increase the slope of the CO2 response below eupnoea and narrow the CO2 reserve despite an accompanying hyperventilation and reduced plant gain [4]. An absence of the normal rise in CO2 (which may be determined by end-tidal measurements using a non-vented oronasal mask or blood gas analysis) at sleep onset is also characteristic of central sleep apnea syndromes [10]. There is recent evidence that patients with “mixed” sleep apnea, that is, showing a substantial central sleep apnea component admixed with the obstructive components, have increased CO2 response slopes below eupnea and a narrow CO2 reserve during sleep but not differences in airway collapsibility [24]. In this study, patients with conventional central sleep apnea were excluded. A substantial component of obstructive sleep apnea severity is mediated by enhanced chemosensitivity [25–30]. Partial responders to upper airway surgery have been reported to have enhanced chemoreflexes [31].
Trait Versus State Components of the Respiratory Chemoreflex
A fundamental question is whether we are dealing with a transient phenomenon or a disease pathophysiology unlikely to remit. There is ample evidence of gene tic /trait effects on respiratory chemoreflexes, although the effects on sleep-respiration were not evaluated in many of these reports [32–50]. Certain mice and rat strains are highly susceptible (or resistant) to periodic breathing following hypoxic exposure [41, 51]. Several knockout mice have altered (usually blunted) hypoxic [52–61] and hypercapnic [62] sensitivity. Evidence for genetic/trait effects in humans include: (1) Individual differences in hypoxic ventilatory sensitivity and its correlation with altitude-induced periodic breathing [63]. (2) Familial clustering of chemoreflex sensitivity [35, 38, 39, 49, 50, 64–67]. (3) Ventilatory instability during sleep onset in healthy individuals being greater in those with high peripheral chemosensitivity [68].
State effects are also important: (1) Hypoxic ventilatory responses to sustained hypoxia including at altitude [69–75]. (2) Increased prevalence of mixed forms of sleep apnea poststroke, in heart failure and increased chemoreflex sensitivity in periodic breathing associated with congestive heart failure or poststroke [16, 76]. (3) A reduction of periodic breathing occurring following cardiac transplantation [77, 78]. (4) An increase of central apneas occuring with age [77–79]. However, chemoreflexes have been reported to be reduced in the elderly, [80–82] so that the mechanisms may be more complicated. (5) Post-tracheostomy central sleep apnea that reduces in severity over time [83, 84]. (6) Recent evidence from a dog model of acute pulmonary venous hypertension suggesting that increases in left ventricular end-diastolic pressure (LVEDP) may reduce CO2 reserve [85]. Of possible relevance, systolic and diastolic cardiac dysfunction is common in severe sleep apnea, [86, 87] but obesity, age, and hypertension are confounders [88]. (7) A 30 % overnight increase in chemoreflex sensitivities having been reported in sleep apnea, whereas in the non-apnea group there was a significant overnight reduction in chemoreflex thresholds (approximately 5 %), without changes in sensitivities [89]. (8) Changes in hypercapnic ventilatory response (HCVR) (reduced sensitivity) having been reported following therapy of obstructive [90–92] but not central sleep apnea [90–92]. Reduction in hypoxic ventilatory response (HVR) but not HCVR was recently reported following 4 weeks positive airway pressure therapy for obstructive sleep apnea [93], while NREM CO2 reserves improves within a month [23].
Polysomnographic Recognition of NREM-Dominant Obstructive Sleep Apnea
The first description of central apneas following continuous positive airway pressure (CPAP) treatment probably came from Marrone et al. who reported the occurrence of central apneas, hypopneas, periodic breathing, and prolonged oxygen desaturations in patients who were started on CPAP. These events occurred in NREM and almost exclusively after arousals or wakefulness periods [97]. The concept was revived in the mid 2000s, with an emphasis of the NREM dominance of disease [98]. The term “complexity” in the context of sleep-disordered breathing was first applied to articulate the problems of conflicting therapeutic principles, with upper airway support for obstructed breathing amplifying respiratory chemosensitivity [99]. It was subsequently proposed that the development of central apnea during titration of CPAP is a “defining characteristic” of complex sleep apnea, with a threshold of 5 or greater central apneas and hypopneas per hour of sleep making up greater than 50 % of all respiratory events [100]. This was then adopted by centers for medicare services and most insurers in the United States. As discussed below, this definition is a core cause of controversy in the field. Though associated with CPAP treatment in the classic description, this phenomenon has also been observed with non-positive airway pressure (PAP) treatments for OSA, including tracheostomy, maxillomandibular advancement surgery, mandibular advancement devices, and other forms of surgical relief [101–103]. Although in most patients, the central apneas emerged only after application after CPAP [99], there is an argument to expand the definition to include emergence of central events in situations other than CPAP therapy.
In this obstructive sleep apnea subset, the demands on the respiratory control systems expose control instabilities and present themselves as a NREM-dominant sleep apnea phenotype [98]. Consequences include a poor response to CPAP, [98] fragmented sleep that is disproportionate to the effects of sleep apnea, and a strong association with anxiety, depression, and hypertension [102]. The polysomnographic phenotype is more reminiscent of the type of sleep apnea seen at high altitude (short cycle time, 25–30 s) [104] than in congestive heart failure [105].
Scoring of respiratory events in sleep apnea patients have traditionally been biased to an obstructive phenotype, though the recent (2012) update of the 2007 American Academy of Sleep Medicine (AASM) guidelines has proposed criteria for scoring “central” hypopneas and short sequences of periodic breathing/Cheyne-Stokes breathing [106]. The guidelines state that “central” hypopneas should not be scored in the presence of flow-limitation, but obstruction is a common feature of central events, [107] even at simulated altitude, [108] the latter being a relatively pure model of chemoreflex-driven sleep apnea. Direct visualization of the upper airway shows collapse at the nadir of the cycle to be common even in polysomnographic “central” respiratory events [109]. Expiratory pharyngeal narrowing occurs during central hypocapnic hypopnea, [110] directly supporting the concept that the presence of flow-limitation alone cannot be used to distinguish obstructive and central hypopneas [108]. Complex sleep apnea as currently “defined” requires a central apnea-hypopnea index ≥ 5/h of sleep with centrally mediated respiratory events constituting ≥ 50 % of all respiratory events during CPAP titration for obstructive sleep apnea, in those who do not fulfill criteria for primary central sleep apnea or periodic breathing on the diagnostic polysomnogram. However, publications of complex apnea did not score “central” hypopneas or periodic breathing. Thus, descriptions of low (< 5 %) persistence of “ complex apnea ” may not be accurate, as this is usually based on scoring central apneas alone, and not central hypopneas or periodic breathing [111, 112]. In our center, at least 15–20 % of obstructive sleep apnea not related to congestive heart failure is recognized to be NREM-dominant. The guidelines for recognition of “Cheyne-Stokes breathing” also require a cycle duration of at least 40 s, but we have shown that even shorter cycle times in the range of 20–25 s is typical of NREM-dominant sleep apnea, [98] reminiscent of high altitude periodic breathing. The most characteristic feature of chemoreflex driving is not the morphology of individual events but NREM-dominance and timing/morphology of sequential events (nearly identical) in a consecutive series of events [113] (Figs. 7.2, 7.3).



Fig. 7.2
Polysomnographic phenotype of chemoreflex driving of sleep apnea. By conventional scoring criteria, these are obstructive respiratory events. However, closer inspection should reveal the following features that are characteristic of NREM-dominant obstructive sleep apnea, which is a presentation of chemoreflex-driven sleep apnea. Note: (1) Self-similar/clone-like respiratory events and pathological oscillations, including hypopneas, electromyographic tone rise and fall, electroencephalographic arousals, and in the finger plethysmographic signal. (2) The respiratory events are short cycle, averaging 20–22 s, strongly reminiscent of high altitude periodic breathing cycle times. Oxygenation is mildly impaired due to the short cycle time of respiratory event—there is not enough time to develop deep desaturations unless cardiopulmonary reserve is impaired. This pattern, if recognized, has an increased risk of positive pressure-induced or amplified respiratory instability

Fig. 7.3
Polysomnographic phenotype of chemoreflex driving of sleep apnea: REM vs. NREM differences. Note (upper, a) short cycle respiratory events with a self-similar morphology and timing, yet “obstructive” by any criteria. When the same subject enters REM sleep (lower, b), the respiratory events “disappear”. This sequence is highly reminiscent of periodic breathing at high altitude. Body position here is unchanged
The ICSD-3 Contribution: Lumping vs. Splitting
The recently published International Classification of Sleep Disorders, 3rd Edition has a new category of treatment-emergent CSA (TE-CSA) [114]. The requirement is for a central apnea index of 5 or more/hour of sleep and the central events greater than 50 % of all scored events. The term complex apnea has been avoided, but the classifications schema allows multiple diagnoses in the same patient, e.g., TE-CAS, OSA and periodic breathing, or periodic breathing, and OSA. This is a “splitting” approach driven by event morphology, which has already been noted to have serious limitations (such that scoring central hypopneas are “optional”), while the term “complex apnea” is a lumping driven by pathophysiology. The merits of the approaches can be debated, but the ICSD-3, based on classic scoring approaches, likely under-recognizes the contribution of enhanced respiratory chemoreflexes to pathogenesis of sleep apnea, which is an important issue as treatment approaches and criteria for treatment success are impacted. More data will settle the issue, but for now the author suggests keeping an open mind, recognizing NREM-dominance, and other tell-tale signs of respiratory chemoreflex activation. These signs include short cycle (30 s or less) of respiratory events, band-like oxygen desaturation (several consecutive desaturation points being identical), periodic breathing regardless of flow-limitation, and EEG arousals in the middle of the respiratory recovery sequence [115]. In general, induction of central apneas and thus meeting the criteria for TE-CSA is not required to recognize strong respiratory chemoreflex driving of sleep apnea.
Quantification of Pathological Chemoreflex Activation During Sleep
How can the effects of an enhanced respiratory chemoreflex be quantified? Daytime testing such as hypoxic and hypercapnic ventilatory responses, measurements of muscle sympathetic nerve activity, serum or 24-h urine catecholamine levels, and heart rate variability measures are not precise enough in individual subjects but can distinguish groups. During sleep, assessment of the output of the respiratory chemoreflexes can take two general approaches. In one approach, that of “provocation,” various maneuvers are used to induce instability during periods of stability [27, 116]. These include the use of proportional assist ventilation, [117, 118] partial obstruction by pressure dial down, [29] and using bilevel-ventilation to induce hypocapnia [10]. An alternate approach is that of “spectral mapping,” which has a focus on low frequency oscillations that dominate light or fragmented sleep in health and disease [119, 120]. The core principle is that when the respiratory chemoreflexes are hyper-responsive, the induced metronomic autonomic/respiratory/hemodynamic oscillations demonstrate a narrow-band feature on spectral analysis (highly limited dispersion of frequencies) [113]. Such patterns are typical of congestive heart failure- associated periodic breathing.
Clinical Implications of Pathological Respiratory Chemoreflex Activation During Sleep
Several studies have estimated the prevalence of complex sleep apnea syndrome (central sleep apnea > 5/hr on CPAP) to be in the range of 13–15 % in the general sleep center patient population [100, 121–124]. Endo et al. reported prevalence of complex sleep apnea in 5 % of Japanese patients, which is lower than reported prevalence in USA and Australia [125]. These patients were mostly male and had maintenance insomnia complaints [124]. They had disturbed sleep and excessive daytime sleepiness [126]. Risk factors for high chemosensitivity forms of sleep apnea (idiopathic central sleep apnea, complex apnea, periodic breathing) include male sex, history of cardiac disease and central sleep apnea on baseline polysomnogram [121]. Although in some patients the apnea-hypopnea index improved over time, many maintained a persistently elevated index with abnormal oximetry. These patients tend to be leaner and sleepier. They also report more air hunger, a sensation of “dyspnea” which may reflect ventilator-patient asynchrony or awakenings at the end of a central apnea, and CPAP mask removal. Other risk factors include higher age, higher baseline apnea-hypopnea index and central apnea index, hypertension, coronary artery disease, stroke and congestive heart failure [127]. In another large retrospective study, severity of sleep apnea and use of opioids were noted to be potential risk factors for complex sleep apnea [112]. However, patients with positive pressure-emergent complex apnea are clinically heterogeneous and one third of patients do not have any risk factors prior to sleep studies [127]. Compliance with CPAP is low and residual machine-detected apnea-hypopnea index high in patients with NREM-dominant sleep apnea.
Natural History of Pathological Respiratory Chemoreflex Activation During Sleep
It is not clear what proportion of complex sleep apnea patients improve over time with continued CPAP treatment. Dernaika et al. in a prospective case-control study documented disappearance of central sleep apnea activity with CPAP in 86 % (12/14) of patients over 2–3 months. However, this finding may not be generally applicable because of small size and their exclusion criteria [128]. Seven of their initial 21 patients were CPAP intolerant or lost to follow-up which suggests possible complex sleep apnea in these patients. Similar findings were reported in a study by Kuzniar et al. [122]. In the largest prospective study performed to date, Cassel et al. reported resolution of complex apnea in some patients, but also reported de novo appearance of the pattern in patients who did not have such characteristics initially [129]. Ultimately, it comes down to the criteria for “persistence”—wave form analysis from current generation positive pressure devices provide us a unique tool to quantify persistent periodic breathing or central apneas and answer this question conclusively. It does seem that persistence of pure central apneas at a high frequency decreases over time [112]. In this study, a strict definition (flat line flow and effort on the polysomnogram) of residual central disease (no scoring of central hypopneas or periodic breathing) was used.
Treatment of Pathological Respiratory Chemoreflex Activation During Sleep
The core goals of treatment of sleep apnea syndromes are normalization of sleep architecture and sleep respiration, including oxygenation and ventilation. Only by addressing upper airway obstruction, respiratory control dysregulation, and sleep fragmentation propensity is successful treatment of HCSA achievable. Each patient is unique in the contribution of these individual elements and the phenotype should drive therapeutic decision making. Weight loss and good sleep hygiene play an important role in the management. Weight loss can reduce the positive pressure requirements and in turn pressure-related hypocapnia and respiratory instability. Sleep onset instability is amplified in patients with apnea in general, and can be especially prominent in those with HCSA syndromes. Poor sleep hygiene or a mismatch between social and biological sleep times (such as in patients with delayed circadian phase syndrome) can increase sleep fragmentation, sleep-wake transitions, and potentially adversely and disproportionately impact HCSA management.
Positive Pressure Therapies—Adaptive Ventilation
More often than not, CPAP is ineffective in patients with HCSA syndromes. (Fig. 7.4). Supporting ventilation during central apneas and stabilizing PCO2 fluctuations has proven successful in treating central and complex sleep apnea. Adaptive Servo-ventilation (ASV) devices are adaptive bilevel positive airway pressure devices introduced in the last decade for treatment of central and complex sleep apnea syndromes. Non-randomized evaluations show the two USA-marketed devices to be equally effective:, the VPAP-AdaptSV® (ResMed Corp, San Diego, CA) and BiPAP-AutoSV® (Phillips-Respironics, Murrayville, PA) [130]. Use of adaptive ventilation in complex apnea patients and central sleep apne/periodic breathing in patients with congestive heart failure (CHF) improved sleep apnea as well as cardiac function parameters [131]. Treatment with ASV is clearly better tolerated than CPAP in patient with TE-CSA or complex sleep apnea more broadly defined, and in patient with congestive heart failure. In these patient groups, adaptive ventilation is more effective in suppressing central apneas and improving oxygenation than CPAP, but residual disease remains high and positive effects on sleepiness, quality of life, and sleep architecture are less impressive [132]. The criteria for success and the respiratory event scoring criteria (usually 4 % desaturation association for hypopneas) can overestimate effectiveness. Two recent reviews provide in-depth summaries of current device algorithms and clinical utilization [133, 134]. These powerful devices allow a range of adjustments, have complex algorithms, and are used in patients with respiratory dyscontrol, where breathing rhythms span the obstructive, central, and ataxic spectrum. Substantial theoretical knowledge, hands-on practice with devices, and skills in recognition of polysomnographic patterns are required for optimal use of these devices (Box 7.1).

The results of the Servo Ventilation in Heart Failure trial (SERVE-HF), with lack of benefit and increase in mortality in patients with a low ejection fraction or Cheyne-Stokes breathing suggest the need to better understand patterns and implications of pressure cycling / patient-ventilator desynchrony and other adverse consequences of adaptive ventilation, including hypocapnia and arousals in vulnerable patients, such as those with reduced ejection fraction.

Fig. 7.4
Periodic breathing/Cheyne-Stokes respiration with obstruction and CPAP effects. Note (upper, a) long cycle periodic breathing in this patient with congestive heart failure . Snoring is seen during respiratory events; narrowing of the airway is common in such patients. There is minor ongoing respiratory effort, enough to drive upper airway vibrations. When CPAP is applied (lower, b), the basic long cycle periodic breathing pattern persists
How to Titrate Adaptive Ventilation
One option is to simply allow the device algorithm to function “wide open”. A physician could request this mode for part of the night. For the Adapt SV that would mean an EPAP minimum of 4 and maximum of 15 cms H2O, and a minimum/maximum pressure support of 5 and 15 cms H2O respectively. For the BiPAP Auto SV that would mean an EPAP minimum/maximum of 4 and 20 cms H2O, a minimum pressure support of 4 cms and maximum pressure support of 25 cms H2O, also setting the inspiratory-maximum pressure of 25 cms H2O. At the end of the titration, the following targets are to be achieved, and thus the technician’s efforts need to be directed to provide the following information.
1.
What is the optimal expiratory support? This may be a challenge in those who have chemoreflex-mediated disease in NREM sleep and obstructive events in REM sleep. In that instance, higher EPAP than needed in NREM sleep or cautious use of narrow range auto-EPAP may be considered.
2.
What is the minimum pressure support that does not trigger respiratory instability? A reasonable default is 3 cms H2O for both adaptive ventilators. If this level of inspiratory support worsens periodic breathing or central apnea and the pattern is maintained despite use of adequate adaptive space, several approaches are open: changing mode of the AV (see next paragraph), non-supine positioning, adding supplemental O2, use of a NV mask, or moving back to CPAP (often with adjuncts—O2, NV mask, non-supine positioning).
3.
What is the optimal mode of the AV? My personal experience in the sleep laboratory is that the degree of device-induced pressure cycling is worse when the Adapt SV is used with a 0 minimum support (the “minimum maximum” is still 5 cms H2O; thus, 3 cms is H2O is preferred as minimum pressure support). However, more titrations need to be done with the mode to be sure of efficacy or lack of it. The BiPAP Auto SV with a 0 inspiratory minimum pressure support does not seems to be a problem, but is not clearly superior.
4.
What should these modes be called? It is arbitrary, but Mode I uses “some” minimum inspiratory pressure support and Mode II uses none. I choose these as above because it is the mode more likely to be actually used.
5.
What is the minimum inspiratory pressure support and what inspiratory pressure support is required to treat inspiratory flow limitation or snoring? The Adapt SV is a default of 5, maximum of 25. The BiPAP Auto SV minimum is zero, the maximum pressure possible is 25 cms H2O. The minimum EPAP for the Auto SV is 4 cms; the minimum EPAP for the Adapt SV is 3 cms.
6.
Is body positioning critical? A subset of patients is absolutely uncontrollable with any current modality, singly or in combination, during unstable supine N2 sleep.
7.
Was supplemental O2 required to stabilize respiratory rhythm?
8.
If a NV approach was used, was additional dead space/Enhanced Expiratory Rebreathing Space required, and what was the ETCO2 at baseline/NREM sleep and REM sleep at optimal settings?
9.
Was sleep fragmentation an independent problem?
10.
I personally think that the auto EPAP function is largely not helpful; the issue is rarely inadequate EPAP or indeterminable EPAP. Moreover, while auto-EPAP is “finding its way” the adaptive function also tries to chase its own targets leading to desynchrony with patient effort. Moderate EPAP (e.g., 5, 6, or 7) is reasonable to start.
11.
Increase pressure support minimum for inspiratory flow-limitation and snoring. If no improvement after 3–4 cms increase, reduce to 3 cms, increase EPAP, and repeat sequence.
12.
Pressure support maximum is increased to increase adaptive “power”—if pressure cycling increases and persists, reduce this component.
13.
When using EERS or O2 with adaptive ventilation—stabilization efforts can alternate between gas/pressure steps.
14.
Rate control: auto function in BiPAP Auto SV and the native rate of Adapt SV are reasonable. Speeding or slowing of the patient’s native rate by 3–4 or more breaths per minute can be a sign of developing desynchrony.
Devices
The ResMed Adapt SV [135–139] provides a baseline degree of ventilatory support. The subject’s ventilation is servo controlled with a high-gain integral controller (0.3 cm H2O per L/min per second, clipped to 4–10 cm H2O) to equal a moving target ventilation of 90 % of the long-term average ventilation (time constant 3 min). If the subject suddenly ceases all central respiratory effort, machine support (i.e., pressure swing amplitude) will increase from the minimum of 4 cm H2O up to whatever is required to maintain ventilation at about 90 % of the long-term average (up to a maximum of 10 cm H2O, reached in approximately 12 s). In the CPAP mode the pressure can be set at 4–20 cm H2O. In the ASV and ASV auto mode EPAP may be set as: 4–15 cm H2O; pressure support (PS): 0–20 cm H2O, but the pressure will not exceed 25 cm H2O. The Auto mode is an auto EPAP. The previous restrictions to PS, such as a minimum support of 3 cm, have been removed with the most recent versions of the software. Algorithm changes will likely continue, to improve patient-ventilator synchrony, such as to dealing with slow breathing rates (current back-up default is 15/minute) and slew rates (maximum rate at which target ventilation can rise, currently 0.01389 L min/sec) [133].
The Philips Respironics BiPAP autoSV Advanced (flow-targeted dynamic bilevel positive pressure ventilator) provides PAP support to sustain upper-airway patency [140–142]. The current version is the “Advanced”, which has auto-CPAP and thus an EPAP minimum and maximum setting. The maximum inspiratory support level is up to 30 cm H2O minus the expiratory pressure. The expiratory pressure automatically adjusts within the available (or prescribed) range (4–25 cm H2O). Expiratory pressures can also be set at fixed levels if desired. The algorithm’s automatic backup rate is based on calculations performed on a moving window of the last 12 spontaneous breaths. The flow-targeted dynamic Bilevel PAP device modulates the maximum and minimum PS above the EPAP as required to maintain a target peak inspiratory airflow: when the device detects normal breathing, flow-targeted dynamic BPAP operates like conventional CPAP by providing the minimal PS; when the patient does not maintain the target peak inspiratory airflow, the device increases the PS up to a maximum IPAP, which can be set by the user. Peak flow is captured on a breath-by-breath basis and is monitored over a moving 4-min window; as one breath is added, the initial breath falls off. At every point within this 4-min period an average peak flow is calculated, and the peak flow target is established around that average and is based on the patient’s needs. The device also provides an automatic backup rate should sustained apnea be detected. To avoid hyperventilating the patient and to promote spontaneous breathing, the target inspiratory flow is set to below the mean inspiratory flow during spontaneous breathing by the patient and the timing of the backup rate begins with time delay and is set to a slower rate than the average respiratory rate of the patient. The current version of the device (BiPAP Auto SV Advanced) allows expiratory pressure relief , where the pressure drops during early expiration in an attempt to improve patient comfort, and rise time (period of time in milliseconds for the pressure to increase to 67 % of the difference from end EPAP to maximum inspiratory pressure) when the pressure relief mode is not enabled [133].
The Weinmann SOMNOvent CR is available in Europe and combines auto and adaptive pressure [143]. The pressure is measured using a pressure sensor, snoring is calculated based on the high-frequency variations of the pressure signal, and the flow is determined based on pressure and blower parameters. The minute ventilation is compared with the average minute ventilation in a moving window and is focused by 50 % on the last 2 min and by 50 % on the previous time. The treatment mode is called anticyclic modulated ventilation. Apneas are defined by a cessation of breathing for more than 10 s. Hypopneas are detected if the minute ventilation is reduced by 40 % for at least two breaths as compared to the average minute ventilation or if the peak flow is reduced by 50 % as compared to the average peak flow. Obstructive and central events are discriminated based on the recognition of flattening of the flow curve and on the reaction on additional PS: obstructions are detected by an increased need for respiratory support with flattening or snoring or by time-cycled breath insufficient to generate flow. The device applies three pressure levels: the IPAP, which represents the pressure level during inspiration; the EPAP, which represents the pressure level in the early expiration; and the end-expiratory positive airway pressure (EEPAP). The EEPAP regulation is based on the cumulative sum of obstructions within a 2-min epoch, not on single events. During periodic hypopneas the algorithm increases the difference between IPAP and EPAP continuously to raise the tidal volume. During hyperventilation the difference between IPAP and EPAP is reduced and can reach CPAP level (zero difference between IPAP and EPAP). During apneas, mandatory breaths are applied automatically. The frequency of these breaths depends on the baseline respiratory frequency of the patients. The physician can choose a manually fixed instead of automatic backup frequency. In addition, the device uses pressure relief during expiration in the course of normal breathing.
Recognition and Scoring of Respiratory Abnormality During Adaptive Ventilation
Scoring respiratory events during adaptive ventilation needs to use the pressure output signal from the ventilator. This is roughly equal and opposite the patient’s abnormality. The flow and effort signals combine patient and ventilator contributions and give a false sense of success. See Fig. 7.5 for excessive “pressure cycling,” which is the response of the adaptive ventilator to ongoing periodic breathing. When pressure cycling persists, sleep fragmentation is usually severe even if respiration is “improved.” This pattern means than periodic breathing pathology is ongoing, necessitating the continued pressure response.


Fig. 7.5
Pressure cycling with adaptive ventilation. The snapshot shows uncontrolled periodic breathing which is unresponsive to all modes of positive pressure, including adaptive ventilation. The ResMed Adapt SV has a characteristic waveform that requires measuring and display of the device’s pressure output (C PRESS in the snapshot above) that is best described as “pressure cycling.” While such cycling is expected and appropriate at the start of implementation of adaptive ventilation, persistence reflects inability of the device to convert periodic breathing to stable breathing. i.e., the device keeps responding to periodic breathing rather than treating the underlying pathology. Supplemental oxygen, non-supine positioning, acetazolamide and a non-vented mask/enhanced expiratory rebreathing space (dead space added to positive pressure ventilation) can all reduce or eliminate pressure cycling. Mild pressure cycling without associated arousals is seen when the adaptive ventilation treatment is successful. The Phillips-Respironics BiPAP Auto SV is somewhat less prone to pressure cycling, and when it does, the saw-tooth pressure waveform is not seen. See Fig. 7.6 for a comparison of CPAP and both adaptive ventilators in the same patient
Challenges of Titration with Adaptive Ventilation
1.
Treating upper airway obstruction can induce or worsen periodic breathing and central apneas, even with these ventilators.
2.
Using the auto EPAP module allows the inspiratory component of the algorithm to induce respiratory oscillations during the EPAP titration period that can in turn cause flow-limitation—thus triggering inappropriate auto-CPAP responses. My default recommendation is to not use this functionality, but manually titrate EPAP. However, others recommend using the auto EPAP mode, and individual sleep laboratories can choose one approach over the other as the initial option.
3.
Excessive minimum inspiratory support will induce hypocapnia and respiratory instability, which will trigger the back-up rate and induce patient-ventilator desynchrony.
4.
Excessive maximum inspiratory pressures may induce pressure discomfort and arousals, besides hypocapnia.
5.
Inadequate minimum or maximum inspiratory pressures will cause inspiratory airflow obstruction and futile triggering of the algorithm.
6.
Inadequate “span” of inspiratory maximum and minimum pressures (the “adaptive space”) will stifle the adaptive algorithm.
7.
With all adaptive ventilation approaches, there is a distinct risk of trading-off airflow and oxygenation/ventilation improvements for sleep quality. This risk is not specific to adaptive ventilators per se, but true for other non-invasive ventilation approaches.
8.
Occasionally, periodic breathing is induced during REM sleep as obstructions or the normal tidal volume fluctuations of the state trigger the adaptive algorithms. The best response is to increase EPAP first, then IPAP, or if there are no arousals, to just leave it be.
9.
One of the most complex functions of adaptive ventilation is the inspiration to expiration switch. Manufacturers use some combination of “human physiological limits,” rate of change in inspiratory flow, detected respiratory rate or other proprietary algorithms.
Minimization of Hypocapnia
The use of supplemental CO2 for hypocapnic central sleep apnea syndromes is old news. That CO2 can stabilize respiration has been known for decades, but high concentrations fragment sleeps by inducing arousals secondary to respiratory stimulation and sympathoexcitation [144, 145]. The key challenge has been delivery of CO2 in a clinically adequate, tolerated, and precise manner. Prevention of hypocapnia is a critical stabilizing factor in sleep respiratory control. “Minimization of hypocapnia” is also physiologically a more appropriate phrase that “induction of hypercapnia” as the latter is utterly unnecessary. The key is holding the CO2 steady and just above the NREM sleep CO2 threshold—protecting the CO2 reserve.
A recent study by Xie et al. is one of the best demonstrations of the power of CO2 modulation in treating sleep apnea syndromes [146]. Twenty-six patients with obstructive sleep apnea (AHI 42 ± 5 events/hour with 92 % of apneas obstructive) were treated with O2 supplementation, an isocapnic rebreathing system in which CO2 was added only during hyperpnea to prevent transient hypocapnia, and a continuous rebreathing system. Each patient’s controller gain below eupnea was measured, as was CO2 reserve, plant gain, and passive upper airway closing pressure. With isocapnic rebreathing, 14/26 reduced their apnea-hypopnea index (AHI) to 31 ± 6 % of control (p< 0.01) (responders); 12/26 did not show significant change (non-responder). The responders vs. non-responders had a greater controller gain, a smaller CO2 reserve but no differences in Pcrit. Hypercapnic rebreathing (+ 4.2 ± 1 mmHg PETCO2) reduced AHI to 15 ± 4 % of control (P < 0.001) in 17/21 subjects with a wide range of CO2 reserve. Hyperoxia (SaO2 ~ 95–98 %) reduced AHI to 36 ± 11 % of control in 7/19 OSA patients tested.
Thus, there is strong evidence from multiple studies over the years that manipulation of arterial CO2 levels might provide an alternative treatment strategy. Addition of a closed volume (space) to exhale increases rebreathing of the exhaled air and results in a rapid increase in arterial CO2 levels and an increased tidal volume and respiratory rate. This is similar to breathing into a bag when trying to treat anxiety-induced hyperventilation. Increased amounts (> 300 mL) manifestly feel uncomfortable from both CO2 retention and volume effects. The concept has been used in mechanical ventilation to reduce hypocapnia for several years and more recently has been successfully used to treat central sleep apnea and Cheyne-Stokes respiration in heart failure. None of these uses combine it with positive airway pressure.
We have shown that keeping CO2 above the apnea threshold with the use of enhanced expiratory rebreathing space (EERS) is an effective adjunct to continuous positive airway pressure therapy in patients with pressure-induced respiratory instability across CSA and periodic breathing phenotypes [102]. The EERS approach is also a useful adjunct to adaptive ventilation, which is described later in this chapter. Enhanced xpiratory rebreathing space is the dead space concept applied to pressure ventilation. Positive airway pressure therapy usually induces mild relative to pretreatment hypocapnia. Central apneas and periodic breathing can be generated when the arterial PCO2 level falls below that required to stimulate respiration. This level is referred to as the apnea threshold. Hypocapnia at or near the apnea or CO2 control threshold destabilizes sleep-breathing control, resulting in periodic breathing patterns of various severities and morphological characteristics. Preventing hypocapnia is a powerful stabilizing influence on sleep-breathing control, regardless of the presence of hypoxia. Atmospheric CO2 levels are near zero, therefore the CO2 levels in the blood are the result of the balance between metabolism in the patient and blow-off during breathing.
The clinical effect of using EERS is gratifying and this approach is now routinely available in our sleep laboratory to use with CPAP or adaptive ventilation, as requested by the physician (Fig. 7.6, 7.7). We described in our original report improved clinical tolerance, compliance and sustained clinical improvement monitored over period of several months by adding EERS to CPAP [102]; since our original publication, we have successfully added EERS to adaptive ventilation in the sleep laboratory and for home use (unpublished). EERS was initially evaluated and used in a subgroup of patients who had stopped using CPAP, and in whom effective treatment was salvaged with the use of EERS. The fundamental idea is that sub-tidal volume dead space would be adequate when the upper airway was also supported, i.e., a synergism between positive pressure and prevention of hypocapnia. Control was achieved with minimal increase in CO2 (0–0.5 mm Hg) which we speculate, judging by the beneficial effects seen within 20–30 s, is due to the effect on carotid chemoreceptors.



Fig. 7.6
Relative efficacy of EERS + CPAP, ResMed Adapt SV or Phillips-Respironics BiPAP Auto SV Advanced in the same patient. The upper panel shows CPAP efficacy, with the end-tidal CO2 held to the mid-1940s. This patient had failed prior positive pressure titrations with induction of central sleep apnea . The middle panel shows relative efficacy of the Adapt SV—pressure cycling (the CPRESS channel) is mild but there are recurrent arousals. The lower panel shows that in this patient, the Auto SV Advanced in the worst. Note severe sleep fragmentation, the flip-flop between patient and machine supported breaths on the CPRESS channel, and the poorly controlled residual apnea

Fig. 7.7
Efficacy of dead space/EERS used with CPAP. From top: 1 Diagnostic polysomnogram component of a split night study. Note classic severe Cheyne-Stokes breathing. 2 Effect of continuous positive airway pressure—cycle time lengthening but no improvement in fundamental respiratory pattern. 3 Addition of 50 cc of dead space/EERS to CPAP using a non-vented mask configuration. Note stabilization of respiration and residual flow limitation. ETCO2 is about 37 mm Hg. 4 Final pressure, 12 cms, with normalization of nearly all flow-limitation and maintained stabilization of respiration. ETCO2 signal shows a partial loss of plateau due to increased leak at the higher pressure
There is strictly no true dead space when this concept is used with mask pressure ventilation. Mixing, turbulence, and leaks ensure variable degrees of effective rebreathing (thus the suggested term enhanced expiratory rebreathing space). There is, however, a reduction in ventilatory blow-off, with remarkable clinical effectiveness. There is no, or minimal, increase in inspiratory CO2 because of the positive pressure-induced washout. One important practical effect of using positive airway pressure with enhanced expiratory rebreathing space is that the respiratory rate seems unchanged, and the subject does not notice any significant discomfort. This is not surprising, given the known effects of positive pressure support on relieving shortness of breath in mechanically ventilated patients. Such symptoms minimization is obviously important when considering use in patients who are already short of breath, such as in heart failure.
The physiological target for titrations with enhanced expiratory rebreathing space is to maintain ETCO2 at the low normal range for sleep. Normal sleep results in an increase of 2–8 mm Hg in ETCO2. With this technique the target is to keep the ETCO2 in the high 30 s to low 40 s, the lower end of normal for patients with hypocapnic central sleep apnea and periodic breathing. Those with central and mixed sleep-disordered breathing show a prominent tendency to hypocapnia during sleep.
The primary monitoring is that of ETCO2 with a mainstream CO2 sensor at the mask. Transcutaneous CO2 may usefully complement end-tidal measures but is not critical for treatment of hypocapnic central/complex sleep apnea. Transcutaneous measurements can have a role in hypercapnic central apnea management. A biocalibration of ETCO2 is done before sleep study (1–2 min rested steady breathing, average final 10 breaths), with a nonvented (NV) mask and 50, 100, and 150 mL added dead space. The biocalibration is used to determine the starting EERS volume and is summarized in Table 7.1 . The CO2 targets are to keep the ETCO2 below 50 mm Hg during sleep and not greater than 5 mm Hg increase from wake. If transcutaneous CO2 is monitored, the target is to keep at less than 50 mm Hg or limit increases to 5 mm Hg from baseline. The latter approach is somewhat approximate, because sleep baseline ETCO2 without an NV mask on the patient, will probably not be known and it is unclear how much time is required for equilibration after sleep onset.
Table 7.1
Determination of starting enhanced expiratory rebreathing space volume
Starting dead space condition | Baseline “wake” ETCO2 a | Suggested starting EERS |
---|---|---|
ETCO2 with NV mask onlya and ≥ 45 mm Hg with 150 mL EERS | ≤ 40 | 50 mL |
ETCO2 with NV mask onlya and ≥ 45 mm Hg with 100 mL EERS | ≤ 40 | 0 or 50 mL |
ETCO2 with NV mask onlya and ≥ 45 mm Hg with 50 mL EERS | ≤ 40 | NV mask only |
ETCO2 with NV mask onlya and ≥ 50 mm Hg with 50 mL EERS | 40–45 | NV mask only |
ETCO2 with NV mask onlya and ≤ 50 mm Hg with 50 mL EERS | 40–45 | NV mask only |
ETCO2 with NV mask onlya and ≤ 50 mm Hg with 100 mL EERS | 40–45 | 0 or 50 mL |
ETCO2 with NV mask onlya and ≤ 50 mm Hg with 150 mL EERS | 40–45 | 0 or 50 mL |
ETCO2 with NV mask onlya | ≥ 45 | Physician approval |
The mask-fitting clinic at the Beth Israel Deaconess Medical Center has modified a large number of masks. Specific masks, methods to convert to NV, and appropriate connectors are available on request (contact Dr. Thomas at rthomas1@bidmc.harvard.edu) and are tabulated with mask-specific PowerPoint files. The nonvented configuration, including the safety valve, adds 60–100 mL of dead space (including the intra-mask space), and this “NV alone” usually means about 60–100 mL of dead space/EERS compared to a vented mask. What this means in practical terms is that adding further EERS is often not necessary any more.
There are two approaches to titration of EERS, “forward” and “backward.” In the forward approach, incremental options are considered with positive airway pressure first with a vented mask, then converting to a nonvented mask, then adding rebreathing space (e.g., 50 mL, then 100 mL, etc.), O2. In the backward approach, maximal stabilization is provided at the outset, starting at 50 mL “dead space” (which seem to be adequate for most) and 2 L/min O2. After obtaining control in REM/stable and unstable NREM sleep, the O2 and CO2 modulations can be progressively withdrawn to assess the minimum requirement. The concept of stable vs. unstable NREM sleep is discussed later in this chapter. Typically, with successful levels of dead space, O2 is not critical but can be a useful backup, because consistent good seals at home are hard to maintain. Obtaining a plateau on the ETCO2 signal ensures effectiveness (i.e., the seal is adequate) and accuracy of the reading. An alternate approach is to simply start with a non-vented mask and no added EERS and titrate as required.
CO2 manipulation can also be done by bleeding CO2 in to the circuit by a more precisely controlled flow-independent method. Thomas et al. reported successful treatment of mixed obstructive and central sleep apnea using a proprietary device, the positive airway pressure gas modulator (PAPGAM), which delivers precisely controlled concentrations of oxygen and CO2. In this small case series, 6 patients with average AHI of 43/h on CPAP improved with reduction of AHI to 4.5/h with addition of 0.5− 1 % using PAPGAM [147]. However, translating this finding to patient care requires the development of a device approved for clinical use.
Oxygen
Nasal O2 has a long history of use to treat central sleep apnea and periodic breathing [148, 149]. Adding oxygen to CPAP may result in better control of complex apnea, with reduction in responsiveness of peripheral chemoreceptors and the loop gain [150]. The limitations include the long term cost and difficulty with reimbursement in “non hypoxic” patients. A recent study in a Veteran’s population showed that O2 has beneficial effects, but the polysomnographic changes were delayed by as much as an hour or more [151]. One change to be aware is that of respiratory event cycle length—which can lengthen with the use of O2. Such a change may “reduce” the respiratory event index but not imply a true stabilization of respiration. Use of O2 also negates use of desaturation link to score hypopneas. It should be noted that hypoxemia levels used to initiate O2 therapy are not the threshold for evaluating supplemental O2—moving saturations from the mid to the high 90s can be beneficial. Use of O2 is off-label for central apnea syndromes. Supplemental O2 can thus usefully be a component of a multi-modal multi-step approach to management of HCSA syndromes.
Sleep Stabilization—“Stable” vs. “Unstable” NREM Sleep
Most patients, including older individuals or those on benzodiazepines/sedative drugs, exhibit periods of sleep that are not scored as NREM stage 3 (N3) by conventional criteria (or any modification thereof) but clearly have ample low amplitude delta frequency waves. Respiration is usually quite stable during this period (functionally, think of it as slow wave sleep), and if recognized, the same precautions as during conventionally scored slow wave sleep apply. Those who study the cyclic alternating pattern (CAP) will recognize this type of “stable” NREM sleep as predominantly non-CAP [152]. In fact, for the purpose of titration, dividing sleep into stable and unstable NREM sleep (or non-CAP/CAP) and REM works well, better than N1–N3 and REM sleep. It may be easier to recognize stable and unstable NREM sleep from non-electroencephalographic signals, such as respiration, heart rate variability, and respiratory amplitude modulation of the electrocardiogram [153]. The stability of this non-CAP or slow wave-like (frequency but not amplitude) sleep can cause a false sense of security (of treatment efficacy); it is also important not to change pressures if this state is recognized (unless obstructions and flow limitation are quite overt). Airflow can look absolutely normal in this state, and then rather abruptly (often within a minute) a switch to being quite abnormal may occur. Such switches of stable/unstable state are spontaneous, and disease or treatment needs to deal with this dynamic.
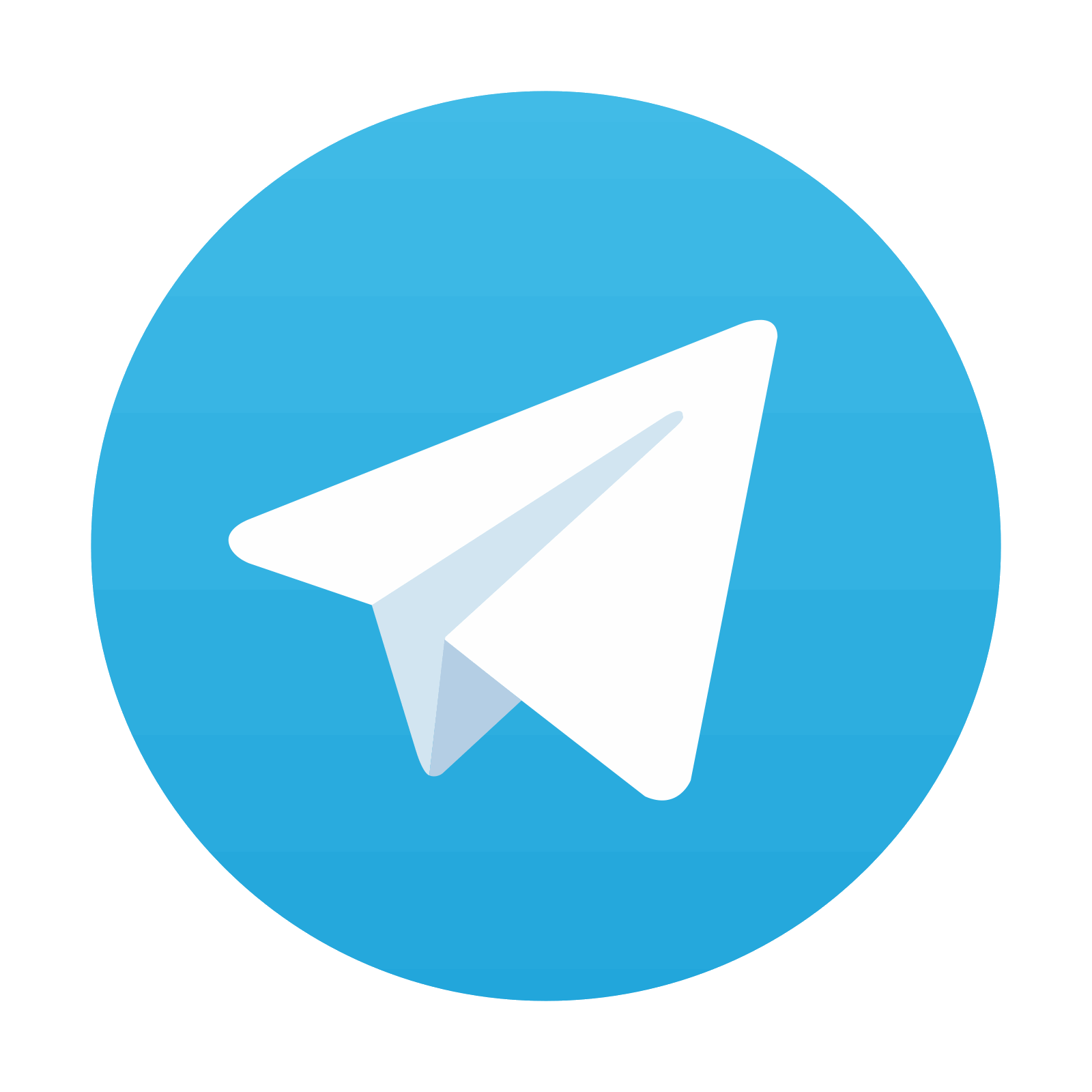
Stay updated, free articles. Join our Telegram channel
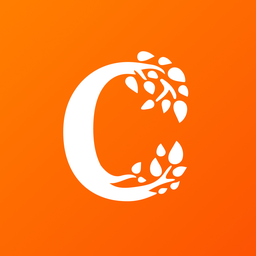
Full access? Get Clinical Tree
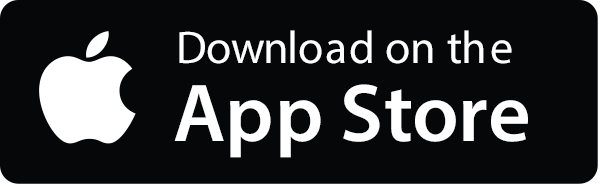
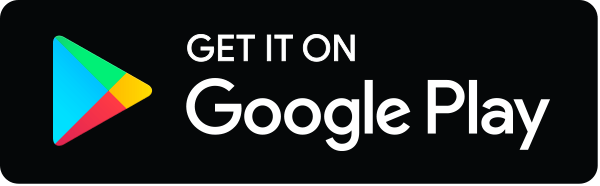