Introduction to Mitral Regurgitation
- Robert A. Levine, MD
Echocardiography has provided the Rosetta stone for deciphering previously unknown dynamic mechanisms that are so critical to understanding pathophysiology, assessing prognosis, and guiding therapy of mitral regurgitation (MR). The mechanism of MR post-MI, for example, was primarily speculative until the advent of echocardiography, with theories ranging from excessive to restricted motion. This was resolved with the echocardiographic demonstration of the incomplete mitral leaflet closure pattern, in which increased leaflet tethering by the papillary muscles restricts leaflet closure. Three-dimensional (3D) echocardiography further differentiated the roles of decreased systolic function and increased tethering in this dynamic force balance applied to the valve. Doppler color flow mapping demonstrated how this force balance creates a orifice area that varies with time and affects quantification. , The puzzle of left ventricular outflow tract obstruction and its attendant MR in hypertrophic cardiomyopathy was similarly clarified by two-dimensional (2D) echocardiography; mechanistic insights are suggesting new therapeutic directions. Echocardiography has clarified the mechanisms of MR in degenerative mitral valve prolapse and provides a dynamic 3D road map in the beating heart for surgical repair. Echocardiography also underlies the phenotypic basis for genetic discoveries and differentiation of potentially different etiologies. Although the basic features of rheumatic mitral stenosis were known from anatomic pathology, echocardiography has provided the noninvasive assessment of features needed to predict benefit of catheter-based therapy. More recently, echocardiography has explored the mechanisms of MR in annular calcification disease. The quantitative strength of echocardiography was first demonstrated in mitral stenosis, with recent advances including rapid, unambiguous quantification of stenotic and regurgitant orifices, using the powerful approach of 3D-guided 2D echocardiography. , Echocardiography has therefore become an integral participant in the evaluation and management of patients with heart valve disease, including MR, and a plays a major role in scientific investigations of underlying mechanisms and improved therapies.
Etiologies and Mechanisms of Mitral Valve Dysfunction
- Benjamin H. Freed, MD
- Wendy Tsang, MD, MS
- Roberto M. Lang, MD
- Wendy Tsang, MD, MS
Diseases that affect the mitral valve are best described by defining the etiology of the disease, the specific lesions caused by the disease, and the dysfunction it creates on the mitral valve apparatus. This “pathophysiologic triad,” first described by Carpentier in the early 1980s, is still extremely useful today in characterizing different types of mitral valve disorders.
Mitral valve etiologies
Mitral valve disease is due to either primary (direct) abnormalities of the mitral valve apparatus or secondary (indirect) causes due to cardiac disease not involving the valve. Examples of diseases that directly affect the mitral valve include congenital malformations such as valve clefts, rheumatic disease, infective endocarditis, trauma, mitral valve annular calcification, valvular tumors, and degenerative diseases. Cardiac diseases that indirectly affect the mitral valve include ischemic and nonischemic dilated cardiomyopathy, hypertrophic cardiomyopathy, and myocardial infiltrative diseases.
Rheumatic mitral valve disease is uncommon in developed countries but continues to be a significant cause of mitral valve disease worldwide. Rheumatic heart disease accounts for about a quarter of all patients with heart failure in endemic countries. Chronic valve disease occurs after one or more episodes of acute rheumatic fever and tends to affect females more than males. Although the mitral valve is involved in almost every case, the aortic and tricuspid valves can also be affected. Younger patients tend to develop pure mitral regurgitation, middle-aged patients more frequently develop mitral stenosis, and older patients usually develop a combination of stenosis and regurgitation.
Degenerative mitral regurgitation, usually associated with mitral valve prolapse, is the most common cause of mitral regurgitation in developed countries. Mitral valve prolapse is an abnormal systolic valve motion of the mitral leaflet into the left atrium (≥ 2 mm beyond the annulus). Three-dimensional echocardiography (3DE) technology has considerably improved the ability of physicians to both diagnose and treat mitral valve prolapse, which results primarily from two distinctive types of degenerative diseases: Barlow disease and fibroelastic deficiency.
Ischemic mitral regurgitation is the pathophysiologic outcome of ventricular remodeling arising from ischemic heart disease and is also a very common cause of mitral regurgitation in developed countries. Ischemic mitral regurgitation occurs in approximately 20% to 25% of patients with myocardial infarction even in the era of reperfusion, and these patients have significantly worse outcomes irrespective of the degree or mitral regurgitation. The resultant volume overload caused by mitral regurgitation worsens myocardial contractility, which, in turn, worsens ventricular dysfunction, eventually leading to heart failure and death.
Mitral valve lesions
No matter the etiology of the mitral valve disease, each disease process frequently results in one or more lesions. For example, dilated cardiomyopathy can result in mitral annular dilatation in what is commonly referred to as functional mitral regurgitation. Degenerative diseases such as Barlow disease and fibroelastic deficiency result in multiple types of lesions, including excess myxomatous leaflet tissue and chordal elongation, thinning, and rupture. Rheumatic heart disease results in commissural fusion, leaflet thickening, and chordal fusion, whereas myocardial infarction can lead to lesions such as papillary muscle displacement, leaflet tethering, and mitral annulus dilatation.
Barlow disease results from an excess of myxomatous tissue, which is an abnormal accumulation of mucopolysaccharides in one or both of the leaflets and many or only few of the chordae. This myxoid infiltration results in thick, bulky, redundant billowing leaflets and elongated chordae, which often lead to bileaflet, multisegmental prolapse. Barlow disease is usually diagnosed in young adulthood, and patients are typically followed for many decades with well-preserved left ventricular size until indications for surgery are met in the fourth or fifth decade of life.
In contrast, fibroelastic deficiency results from acute loss of mechanical integrity due to abnormalities of connective tissue structure and/or function. It usually results in a localized or unisegmental prolapse due to elongated chordae or flail leaflet due to ruptured chordae. Patients most commonly present in the sixth decade of life with a relatively short history of mitral regurgitation. This entity is the most common form of organic mitral valve disease for which mitral valve repair surgery is required.
There is considerable overlap between these two entities, and it is difficult to reliably distinguish them based on either the gross or histologic appearance of the valve. 3DE has the ability to differentiate between Barlow disease and fibroelastic deficiency. When 3DE quantitative parameters were used to differentiate between patients with and without degenerative mitral valve disease, billowing height and volume were the strongest predictors for the presence of degenerative mitral valve disease. Furthermore, 3DE billowing height with a cutoff value of 1.0 mm differentiated between normal and degenerative disease without overlap, and 3DE billowing volume with a cutoff value of 1.15 mL differentiated between Barlow disease and fibroelastic deficiency without overlap. Some valves may represent a forme fruste of Barlow disease and will demonstrate myxoid infiltration on subsequent histological examination ( Fig. 114.1 ).

Classically, ischemic mitral regurgitation was thought to develop due to posteromedial papillary muscle dysfunction given this muscle’s dependence on a single blood supply. In the past decade, however, multiple 3DE studies have shown that papillary muscle dysfunction is not responsible for ischemic mitral regurgitation. In fact, there is a wide spectrum of geometric distortions secondary to left ventricular remodeling that result in this type of valve dysfunction. The observations provided by 3DE have helped reshape our understanding of ischemic mitral regurgitation.
The mitral valve is dynamic and changes from a saddle shape (hyperbolic paraboloid) during systole to a flatter configuration during diastole. During systole, there are competing forces acting on the mitral valve leaflets. Increased left ventricular pressure acts to push the leaflets toward the left atrium while tethering forces from the chordae act to pull the leaflets in the direction of the left ventricle. The saddle-shape morphology is believed to balance these forces by optimizing leaflet curvature and, thus, minimizing mitral leaflet stress. In the setting of a myocardial infarction and resultant left ventricular remodeling, an outward and apical displacement of the posteromedial papillary muscle occurs, which tethers the mitral valve leaflets into the left ventricle, restricting their ability to coapt effectively at the level of the mitral annulus.
This mitral leaflet tethering is a major contributing factor to the development of ischemic mitral regurgitation. Two-dimensional echocardiography has been extensively used to calculate the mitral valve tenting area and tenting length; however, studies have shown that the asymmetry of these single-plane measurements is commonly inaccurate compared with intraoperative findings. 3DE overcomes this limitation by providing more accurate and reproducible measurements.
In one of the first studies to examine leaflet tethering with 3DE, patients with severe mitral regurgitation were shown to have significantly larger tethering lengths and tenting volumes compared with control patients. Furthermore, this study found that the leaflet site where peak-tenting occurred was different in each individual. This suggests that different chordae are involved in the disease process.
Conformational changes of the mitral valve annulus also contribute to the development of ischemic mitral regurgitation. Multiple studies have shown that the annulus dilates and flattens, becoming essentially adynamic throughout the cardiac cycle. , In addition, 3DE imaging has revealed more subtle anatomic changes such as greater dilation in the anteroposterior dimension and greater overall dilation and flattening in anterior compared with inferior infarcts. , In addition, 3DE has been used to evaluate the dynamic changes in mitral valve annular surface area and annular longitudinal displacement throughout the cardiac cycle. It has been demonstrated that the mitral annular surface area is larger and the annular pulsatility and displacement lower in patients with ischemic mitral regurgitation. As the mitral annulus enlarges, it loses its motility, becoming progressively unable to modify its shape throughout the cardiac cycle.
One of the most intriguing findings by 3DE is that, while leaflet tethering and annular geometric changes drive the development of ischemic mitral regurgitation, leaflet growth occurs in an attempt to compensate for the decrease in leaflet coaptation. In one of the earliest studies to examine this phenomenon, Chaput and colleagues found that leaflet area increased by 35% in patients with left ventricular dysfunction. In fact, 2 months after a myocardial infarction, tethered leaflet area and thickness were shown to be significantly increased compared with nontethered leaflets. Studies using molecular histopathology showed that this leaflet growth might be due to an increase in α-smooth muscle actin in tethered leaflets indicating endothelial-mesenchymal transdifferentiation.
A recent 3DE study examined the interaction between leaflet tethering, annular dilation and flattening, and leaflet elongation. The authors measured multiple variables including tenting length and volume, total leaflet area, total annular area, and coaptation length and area. They demonstrated that mitral leaflet coaptation decreases proportionally to the increased displacement of the papillary muscles, despite the presence of compensatory increased total leaflet area. In addition, the ratio of total leaflet area to total annular area required to ensure proper coaptation in mid-systole was decreased in patients with severe mitral regurgitation compared to patients with only mild mitral regurgitation. Indeed, coaptation area was the strongest determinant of mitral regurgitation severity. The question as to why some patients develop sufficient leaflet growth while others do not remains unknown.
Mitral valve dysfunction
All of these lesions lead to mitral valve dysfunction. Instead of classifying this dysfunction as simply mitral valve stenosis or regurgitation, Carpentier developed a classification scheme to aid in the surgical strategy based on the type of leaflet motion ( Fig. 114.2 ). Patients with mitral annular dilatation or leaflet perforation usually have normal leaflet motion and are categorized as type I dysfunction ( Fig. 114.3 ). Type II dysfunction includes patients with prolapse and flail (excessive motion of the leaflet margin above the plane of the annulus) due to excessive and redundant leaflet tissue or chordal rupture, respectively ( Fig. 114.4 ). Leaflet restriction during valve closure due to fusion of various components of the mitral valve apparatus is defined as type IIIa dysfunction ( Fig. 114.5 ), whereas leaflet restriction during valve opening resulting from leaflet tethering is defined as type IIIb dysfunction.




It is important to emphasize that the different components of the pathophysiologic triad are not mutually exclusive and can be clinically combined in different ways. For example, the typical lesions seen in type IIIa dysfunction can also occur in conjunction with the lesions of type II dysfunction. Type IIIb dysfunction is the result of ventricular remodeling, with the primary lesion being leaflet tethering due to papillary muscle displacement as occurs in ischemic mitral regurgitation. Associated annular dilatation is a common finding in patients with chronic degenerative mitral regurgitation, but the classification of dysfunction should differentiate the primary lesion causing the regurgitation (i.e., chordal rupture) from secondary lesions (i.e., annular dilatation).
Mitral regurgitation is a very common disease that can lead to substantial morbidity and mortality. Understanding the multiple underlying causes of this disease and their respective mechanisms of valvular disturbance is crucial to choosing the appropriate treatment.
Mitral Valve Prolapse
- Wendy Tsang, MD, MS
- Benjamin H. Freed, MD
- Roberto M. Lang, MD
- Benjamin H. Freed, MD
Mitral valve prolapse (MVP) is the most common cause of mitral regurgitation in developed countries. It is also known as degenerative or myxomatous mitral valve disease and comprises a spectrum, with its mildest form known as fibroelastic deficiency and its most severe as Barlow disease ( Table 115.1 ). In this chapter we will discuss the etiology, diagnosis, and management of MVP.
Differentiating Characteristics | Barlow Disease | Fibroelastic Deficiency |
---|---|---|
Pathology | Excess leaflet tissue due to accumulation of mucopolysaccharides | Loss of mechanical integrity due to impaired production of connective tissue |
Typical age at diagnosis | Younger (< 40 years old) | Older (> 60 years old) |
Duration of disease | Years to decades | Days to months |
Physical exam | Midsystolic click and late systolic murmur | Holosystolic murmur |
Leaflet involvement | Multisegmental | Unisegmental |
Leaflet lesions | Leaflet billowing and thickening | Thin leaflets with thickened involved segment |
Chordal lesions | Chordal thickening and elongation | Chordal elongation and chordal rupture |
Carpentier classification | Type II | Type II |
Type of dysfunction | Bileaflet prolapse | Prolapse and/or flail |
Complexity of valve repair | More complex | Less complex |
Etiology
The form of MVP known as fibroelastic deficiency occurs when there is an acute loss of mechanical integrity due to abnormalities of the mitral valve connective tissue structure and/or function. This typically results in a localized, single-segment prolapse due to elongated chordae or flail leaflet due to ruptured chordae ( Fig. 115.1 , A ). Patients most commonly present in the sixth decade of life with a relatively short history of mitral regurgitation (MR).

In contrast, Barlow disease results from an excess of myxomatous tissue, secondary to abnormal accumulation of mucopolysaccharides in multiple scallops of one or both leaflets and many or only few of the chordae. This myxoid infiltration leads to thick, bulky, redundant billowing leaflets and elongated chordae. Overall, this form is best recognized by imaging and at surgery as bileaflet, multi-segmental prolapse (see Fig. 115.1 , B ). Because of this chronic process, Barlow disease is usually diagnosed in young adulthood, and patients are typically followed for many decades with mild mitral regurgitation and well-preserved left ventricular size until indications for surgery are frequently met in the fourth or fifth decade of life.
Diagnosis
The specific diagnosis of fibroelastic deficiency versus Barlow disease is often difficult to definitively determine by either the gross or histologic appearance of the mitral valve because of the overlap of these diseases. Some valves that appear to be secondary to fibroelastic deficiency may actually represent a forme fruste of Barlow disease and will demonstrate myxoid infiltration on subsequent histological examination. However, preoperative differentiation of the extent and location of mitral involvement is crucial for surgeon-specific referral, determination of optimal surgical strategy, and postoperative outcome. The lesions resulting from Barlow disease are complex and frequently require superb surgical skills to achieve a successful repair, whereas lesions resulting from fibroelastic deficiency are more localized and can typically be undertaken with simple repair techniques successfully achievable by most cardiothoracic surgeons. Poor presurgical planning may lead to either unsuccessful repair or conversion to valve replacement with less favorable outcomes in patients with complex valvular diseases. ,
Three-dimensional echocardiography (3DE) has greatly improved the ability to diagnose and treat MVP. Multiple studies have shown that 3DE is superior to 2DE in accurately diagnosing MVP when compared with surgical findings. , 3DE is less operator-dependent and more reproducible than 2DE at any expertise level. As well, 3D transesophageal echocardiography (TEE) correctly identified prolapse in 92% of patients versus 78% of patients using 2D TEE. 3DE has also improved quantification of the associated regurgitation.
Parametric maps transform the 3DE images of the mitral valve into color-encoded topographic displays of mitral valve anatomy ( Fig. 115.2 ). The color gradations on the parametric maps indicate the distance of the leaflet from the mitral annular plane toward the left atrium. The use of these maps has been demonstrated to improve the diagnostic accuracy and reproducibility of interpretation by novice readers, when compared to 2D echocardiography. More recently, these maps have been reported to improve the differentiation of mitral leaflet billowing from prolapse while accounting for the saddle shape of the mitral annulus. On 2D echocardiography, mitral valve prolapse is identified if the mitral leaflet tip is above the annular plane at end-systole. In contrast, mitral valve billowing is identified if the leaflet body protrudes above the annular plane but the leaflet tip remains at or below the annulus at end-systole. With 3DE, leaflet billowing can be identified on 3D parametric maps, when the line of coaptation between the anterior and posterior leaflets is intact and the region of maximal leaflet excursion into the left atrium does not extend to the coaptation zone ( Fig. 115.3 ). Similarly, leaflet prolapse is identified when the line of coaptation is disrupted by gaps and the region of maximal leaflet excursion extends to the zone of coaptation.


In addition to improving visual assessment of the valve, these maps also allow quantitation of mitral valve parameters, such as leaflet height and annular area. This has provided additional factors for consideration in differentiating Barlow disease from fibroelastic deficiency. 3DE quantification of billowing height with a cutoff value of 1.0 mm can differentiate between normal valves and MVP without overlap, whereas 3DE billowing volume with a cutoff value of 1.15 mL can differentiate between Barlow disease and fibroelastic deficiency. Of note, these measurements were found to be highly reproducible.
Mitral Valve Repair
3DE quantification has also been applied in MVP patients undergoing mitral valve repair. Prolapsing height and anterior leaflet surface area derived from 3DE parametric maps can accurately predict surgical repair complexity, irrespective of MR etiology. Also, with 3D quantification, it was found that (1) 3DE mitral annular dimensions were accurate and reproducible, compared with direct intraoperative measurements; (2) patients with MVP have significantly larger annular dimensions than controls during diastole; (3) control patients have early-systolic anteroposterior and area contraction, increased annular height, larger saddle shape depth, and unchanged intercommissural diameter, whereas patients with MVP have mostly unchanged annular dimensions, albeit with significant intercommissural dilation; and (4) after repair, the annulus is smaller in MVP patients but continues to lack systolic saddle-shape accentuation.
Overall, transesophageal 3DE provides in greater detail information regarding the pathomorphologic changes to the mitral valve, allowing a tailored rather than standard approach, in which a preestablished operation is performed according to the Carpentier classification system. This is an especially important change in the surgical approach to myxomatous mitral valve disease, because patients often have more than a single mechanism of MR, which limits the utility of the Carpentier classification system.
3DE has also highlighted the different conformational changes that occur between Barlow disease and fibroelastic deficiency after mitral valve repair. Not surprisingly, the annular diameters and mitral valve area were significantly reduced for both disease entities postrepair. However, the mitral valve annulus was found to be larger in patients with Barlow disease, which is consistent with the different mean sizes of the implanted prosthetic rings. In addition, the greater reduction in posterior leaflet area in patients with Barlow disease compared with fibroelastic deficiency is in agreement with the higher rate of posterior leaflet resection and sliding performed for this type of degenerative disease.
The propensity for developing systolic anterior motion after mitral valve repair is, in part, dependent on the degree of mitro-aortic and septo-aortic angles, presence of excess tissue, and displacement of mitral coaptation line toward the posterior leaflet, all of which can be well characterized by 3DE. By quantifying the extent of the excess anterior and posterior leaflet length, surface area, and billowing volume before and after surgery, 3DE analysis helps identify patients who are most at risk for developing systolic anterior motion.
Summary
MVP is a spectrum that ranges from fibroelastic deficiency to Barlow disease. 3DE has improved diagnosis by improving not only accuracy of lesion localization but also quantification of the associated MR. These improvements in turn have favorably altered mitral valve surgical repair techniques and percutaneous interventions.
Quantification of Mitral Regurgitation
- Wendy Tsang, MD, MS
- Benjamin H. Freed, MD
- Roberto M. Lang, MD
- Benjamin H. Freed, MD
Echocardiography is the primary modality for evaluating patients with mitral regurgitation (MR) and should include assessment of the severity, mechanism(s), reparability, prognosis, and hemodynamic consequences on the left ventricle (LV) and left atrium (LA). This chapter will focus on the quantitation of MR. Box 116.1 lists the methods used for estimating the severity of MR. Established methods derived from two-dimensional (2D) echocardiographic data include color and spectral Doppler. However, it is well known that these methods are imperfect because the complex geometry of the mitral orifice results in a three-dimensional (3D) regurgitant jet that is poorly assessed by 2D imaging. The development of three-dimensional echocardiography (3DE) has improved the assessment of MR with improved calculation of the effective regurgitant orifice area (EROA) by vena contracta area or proximal isovelocity surface area (PISA) and true 3D anatomical regurgitant orifice area (AROA) (see Box 116.1 ). This chapter covers both the 2D and 3D methods used to quantify MR and includes supporting signs such as left atrial and left ventricular size.
Semi-quantitative
- •
Color jet area
- •
Signal intensity
- •
Antegrade flow across the mitral valve
- •
Pulmonary venous flow pattern
- •
V-wave cutoff sign
Quantitative Methods
- •
Vena contracta
- •
PISA: EROA and regurgitant volume
Indirect (supporting) signs
- •
Left atrial size
- •
Left ventricular size
EROA , Effective regurgitant orifice area; PISA , proximal isovelocity surface area.
Definition of severe mitral regurgitation
Current guidelines state that EROA ≥ 0.4 cm 2 , regurgitant volume ≥ 60 mL, and regurgitant fraction ≥ 50% constitute severe mitral regurgitation in patients with primary regurgitation. , In contrast, for patients with secondary mitral regurgitation, cutoffs are EROA ≥ 0.2 cm 2 , regurgitant volume ≥ 30 mL, and regurgitant fraction ≥ 50%. This difference in cutoffs exists because PISA measurement by 2D transthoracic echocardiography in patients who have secondary MR results in an underestimation of the true effective regurgitant orifice because of the crescent shape of the proximal convergence.
Hemodynamics underlying the assessment of mitral regurgitation
All 2D and 3D echocardiographic measurements used to evaluate MR severity are based on the Gorlin hydraulic orifice equation
Vreg = AROA × Cd × MPG × T
MPG
is the square root of the systolic mean pressure gradient between the left ventricle and the left atrium, and T is the duration of mitral regurgitation. This formula can be further simplified to
Vreg = EROA × VTI
First, simplification of the Gorlin equation can occur because EROA and AROA are not equivalent. EROA is related to AROA through the discharge coefficient (Cd), which corrects for energy loss and contraction of the flow stream as it passes through the AROA. The discharge coefficient generally falls between 0.80 and 0.85, and its value is affected by orifice geometry, flow and fluid viscosity. Because it is not equal to 1.0, the EROA is 15% to 20% smaller than the AROA. It must be noted, however, that AROA (and, by default, EROA) is one of the most important component in evaluating MR severity. However, AROA is dynamic and load-dependent, which creates difficulties in obtaining an accurate measurement of AROA. This is because the mechanism of MR is often dynamic. For instance, in degenerative MR, the AROA will decrease during mid-systole and can vary due to patient hemodynamics or loading conditions.
Second, the velocity time integral (VTI) of the MR jet is mathematically identical to the product of the square root of the mean peak gradient and the duration of MR during systole. These two components that make up the VTI of the MR jet explains why hemodynamic conditions such as patient blood pressure and duration of MR affect accuracy of MR severity assessment. The duration of MR is important, especially in mitral valve prolapse, in which late systolic MR may lead to overestimation of severity. This occurs mainly in single-frame measurements such as jet area, vena contracta width, or proximal isovelocity surface area. A large jet that appears late in systole may result in a large EROA but a small regurgitant volume. When this discrepancy occurs, quantification can help determine the true severity.
With the simplified formula [Equation 2], regurgitant volume can be measured from 3D echocardiography and EROA can be calculated by dividing regurgitant volume by VTI and vice versa. Although there are strengths and weaknesses to measuring EROA and then deriving regurgitant volume, or measuring regurgitant volume and then deriving EROA, the ability to measure both EROA and regurgitant volume is useful in determining MR severity.
Anatomy
All assessments of mitral regurgitation should include assessment of mitral valve anatomy, which includes annular and subvalvular structures such as the chordae and papillary muscles, as well as left ventricular function and left atrial size. If possible, the mechanism of MR should be provided. Severe MR rarely occurs when the entire mitral apparatus and left ventricular structure are normal. Additionally, left ventricular function and left atrial size provide clues to the chronicity of MR. Finally, LV dysfunction and increased body-surface area indexed LA volume at diagnosis have been associated with worse outcomes in patients with MR. The role of LV dilation has led to guidelines recommending that patients with severe MR and LV end-systolic diameter > 4.0 cm or ejection fraction ≤ 60% be considered for surgery.
Two-dimensional color doppler
Color Doppler depicts the spatial distribution of velocities within the imaging plane rather than flow velocities. Thus it is a useful method to determine the presence or absence of MR. However, visual assessment is unreliable in determining severity. This is because equipment manufacturers, instrument settings, and hemodynamic variables can affect the images that are obtained. Thus the same jet may appear differently on different echocardiographic machines. Therefore, reliance on visual jet assessment is not recommended. Assessment of 2D color Doppler imaging includes jet area, ratio of jet area to LA area, vena contracta width ( Fig. 116.1 ), and calculation of EROA by the PISA method.

Two-Dimensional Color Doppler Jet Area
It had formerly been advocated that color MR jet area either alone or indexed to LA area (see Fig. 116.1 , A , B ) could be a useful method to grade severity. Although assessing color jet area is typically the starting point for quantitating MR, it by no means should be the sole technique. The color jet area should not be mistaken for the MR volume. Unfortunately the MR volume (a more direct measure of the degree of MR) is not the only factor that determines the color jet area. Numerous technical, physiologic, and anatomic factors affect the size of the regurgitant jet area. Some of these are listed in Box 116.2 .
- •
Regurgitant volume
- •
Velocity of regurgitation (driving pressure)
- •
Size and compliance of the left atrium
- •
Central versus wall-hugging jets
- •
Regurgitant orifice size and shape
- •
Influence of co-existing flow streams
- •
Heart rate
- •
Technical factors:
- •
Gain setting
- •
Scale
- •
Frame rate, sector size
- •
Color flow algorithms
- •
Echo machine
- •
Thorough evaluation of the MR color jet area is necessary to demonstrate its full extent. This requires careful angulation or tilting of the transducer from multiple views, including off-axis views. The standard, recommended technique is to use a Nyquist limit of 50 cm/sec to 60 cm/sec and a gain setting that barely eliminates color speckles from cavitary regions with non-moving flow. Eccentric, “wall-hugging” jets will appear smaller than centrally directed jets of similar volume of regurgitation because these jets are unable to entrain blood on all sides. , Centrally directed jets may appear larger because they entrain volume on all sides. Color flow area also varies with the driving pressure across the mitral valve (the difference between the systolic blood pressure and the LA pressure). Thus color Doppler jet area should not be the only parameter used to assess MR severity.
Two Dimensional Vena Contracta Width
The vena contracta is the narrowest, highest-velocity region of jet flow that occurs between the proximal flow convergence region and the actual regurgitant jet before expanding into the left atrium (see Fig. 116.1 , C ). It typically forms a “neck” and is located at or just downstream from the regurgitant orifice. If the jet is symmetrical and circular, then the vena contracta width will reflect the EROA. Ideally, the vena contracta should be measured in the parasternal long-axis view perpendicular to mitral leaflet closure. , The apical view is not recommended because of limitations in spatial resolution. In particular, measurement from the 2-chamber view may be misleading because this view is parallel with the coaptation line. Jets secondary to leaflet tethering and annular dilation are known to be asymmetrical, typically longest along the coaptation line, and therefore measurement in this view will greatly overestimate the severity of mitral regurgitation.
Vena contracta measured by transthoracic and transesophageal echocardiography is very reliable, with a width < 0.3 cm indicating mild MR and a width ≥ 0.7 cm specific for severe MR. Intermediate widths between 0.3 and 0.7 cm do not necessarily distinguish moderate MR from mild or severe MR, and further quantitation is needed. One strength of the vena contracta measurement is that it is reliable for both central and eccentric jets. , Another strength is that it is independent of flow rate. However, vena contracta width may vary during systole because of dynamic changes in the AROA. Limitations of vena contracta include the need to align the imaging plane perpendicular to the vena contracta and inaccuracies that occur when the vena contracta is not symmetrical. Also common to all single frame measurement techniques, overestimation may occur with late systolic prolapse because the leak does not occur throughout systole.
Two-Dimensional Proximal Isovelocity Surface Area Method
To understand the PISA method, it is necessary to understand the underlying hydrodynamic principle. As flow approaches a circular orifice, concentric hemispheric shells of increasing velocity and decreasing surface area are formed proximal to the orifice. With color Doppler imaging, the hemispheric shells proximal to the regurgitant orifice area can be identified and the radius measured. The radius is then used to calculate the surface area of the hemisphere (2πr 2 ). The product of the surface area of the hemisphere and the aliasing velocity is the flow rate in mL/sec. If the maximal PISA radius occurs at the same time as the peak regurgitant velocity, then the maximal EROA is
EROA = 6.28 r 2 × Va / PkVreg
There are four major caveats to this method. First, the PISA jet must be acquired properly. The aliasing velocity should be adjusted so that a well-defined hemisphere is shown. Whether it is on transthoracic or transesophageal echocardiography, shifting the baseline towards the direction of the MR jet rather than simply lowering the aliasing velocity to a predetermined set point achieves this ( Fig. 116.2 ). Second, the base of the hemisphere should be flat (i.e., 180°). If it is not, then a correction should be performed, which is the ratio of the angle formed by the walls adjacent to the regurgitant orifice and 180°. Third, the orifice shape affects the shape of the PISA. A round orifice results in a hemispheric PISA, whereas an eccentric orifice may result in an elliptical or prolate flow convergence. The PISA calculation assumes a hemispheric shape. With a non-circular orifice, PISA will underestimate EROA. Fourth, it is necessary for the continuous wave Doppler signal to be aligned with the regurgitant jet because poor alignment will result in an underestimation of velocity and an overestimation of EROA by PISA. Tips for calculating EROA by the PISA method are listed in Box 116.3 . It is probably not necessary to obtain PISA if the degree of MR is trace or mild, or if MR is obviously severe when obtained by other methods.

- 1.
Zoom on the mitral valve from an apical view.
- 2.
Shift the color baseline down (in the direction of the regurgitant jet) to increase the PISA hemisphere, facilitating measurement of its radius.
- 3.
Obtain multiple beats-per-clip and choose only a hemispheric PISA “mushroom” (dome).
- 4.
Measure the radius from the valve tips to the first aliased velocity at the red (usually yellow)-blue interface. This occurs in mid- to late systole.
- 5.
The Doppler beam should be parallel to MR jet to accurately measure the peak MR velocity.
MR, Mitral regurgitation; PISA, proximal isovelocity surface area.
Two-Dimensional Volumetric Methods
Flow rate and stroke volume at the mitral and aortic valve annuli can be obtained using pulsed Doppler VTI recordings and 2D measurements through the following formula:
SV = CSA × VTI

An alternative method to calculating regurgitant volume is to determine stroke volume using the difference of LV end-diastolic and end-systolic volumes. One limitation to this technique is that 2D transthoracic echocardiography tends to underestimate stroke volume because of LV foreshortening of the apex and difficulties delineating the endocardial border.
Three-dimensional echocardiography
The development of 3D echocardiography (3DE) has led to improvements in quantifying MR through the ability to assess the 3D nature of the MR jet. 3DE has not only increased understanding of the shape of the MR jet but also allowed evolution of 2D methods to provide more accurate assessment of MR. The effect of 3DE on the measurement of vena contracta, PISA, AROA, and stroke volume will be discussed in the following paragraphs.
Three-Dimensional Vena Contracta Area
Direct assessment of the vena contracta by 3DE reveals significant asymmetry of the vena contracta area in MR. This is best demonstrated in patients with ischemic MR, in which 3DE examination reveals the vena contracta area to be eccentric rather than circular. In facts, studies have demonstrated that less than 10% of patients have truly circular vena contracta areas. This highlights why EROA is underestimated by 2D single-plane vena contracta width measurements. The vena contracta width on 2D echocardiography is highly dependent on where the cut-plane passes through the vena contracta, and accordingly will be wider in some views than in others ( Fig. 116.4 ). 3DE allows direct visualization of the vena contracta area, which can then be measured. This has been demonstrated to be not only a reliable technique but also an accurate one. Multiple studies have compared vena contracta area measurement by 3DE to various 2D quantitative parameters and have consistently reported that accuracy and reproducibility for MR severity is superior when 3D vena contracta area measurements are used. A 3D vena contracta area cutoff of 0.41 cm 2 is 82% sensitive and 97% specific in differentiating moderate from severe MR, regardless of whether MR etiology is ischemic or degenerative.

Three-Dimensional Proximal Isovelocity Surface Area
Calculation of EROA from a 2D echocardiographic study inputs the radius into a formula that assumes that the proximal flow convergence region is hemispherical and that the regurgitant orifice is circular. However, 3D computational fluid dynamics models have demonstrated that as the mitral regurgitant orifice gets larger, the convergence region becomes spheroidal (flattened) near the orifice and ellipsoidal (elongated) farther from the orifice. Thus the use of a formula that assumes an underlying hemispheric shape is frequently inaccurate. However, when hemiellipsoidal formulas are substituted into 2D echocardiographic formulas for calculating EROA, a more accurate estimation of the effective regurgitant orifice area in both in vitro and clinical studies has been achieved. ,
To improve the accuracy in calculating EROA, some studies have used multiplanar reconstruction techniques to obtain the largest PISA radius from a 3D color Doppler data set. Unfortunately, although this allows a more accurate assessment of the EROA, it still relies on geometric assumptions regarding the proximal flow convergence region. A solution to this would be to not measure EROA from 2D PISA recordings but to directly measure the PISA from the 3D data set. This has recently been accomplished with the development of software that allows direct tracing of the convergence zone in multiple radial imaging planes with reconstructions of the total surface area, thus eliminating the need for geometric assumptions ( Fig. 116.5 ). ,

Three-Dimensional Anatomic Regurgitant Orifice Area
Because 2D planimetry of the EROA in patients with MR is inaccurate due to the complex, non-planar 3D geometry of the orifice, 3D AROA measurement may provide a reasonable alternative to determine the severity of MR. The AROA is accomplished by direct visualization of the mitral valve en face ( Fig. 116.6 ).

There are several methods for determining the AROA. One method requires manual tracing of the leaflet edges within a 3D dataset. This method demonstrates good correlation with 2D PISA-derived EROA but has better reproducibility. Another method that does not fully account for the 3D shape of the AROA is to use multiplanar analysis of the 3D dataset of the mitral valve to identify the AROA and obtain a planar measurement of it.
Three-Dimensional Mitral Inflow and Left Ventricular Outflow Tract Stroke Volume
As an alternative to estimating EROA or measuring AROA, the severity of regurgitation can be also determined by quantitating the regurgitant volume. Although this can be measured using 2D echocardiography, 3DE is superior because it allows stroke volume quantification without geometric or flow profile assumptions or reliance on single-plane measurements. This technique uses 3D color Doppler data within a region of interest to calculate stroke volume. Studies have demonstrated the accuracy of 3D-derived left ventricular outflow and mitral inflow stroke volume measurements. , Recently, a new method for quantifying mitral valve regurgitant volume has been developed using a single 3D transthoracic echocardiography volume data set to obtain both 3D-derived left ventricular outflow and mitral valve inflow stroke volumes. This method reported that left ventricular outflow and mitral valve inflow stroke volume measurements, using real-time 3DE, were significantly more accurate and reproducible than those obtained with 2D echocardiography. Additionally, this technique was highly feasible, and post-processing data required less than 1 minute.
Limitations of Three-Dimensionally–Derived Quantitative Measurements for Mitral Rergurgitation
Althought there are many advantages to using 3DE to assess mitral regurgitation, there are also limitations ( Table 116.1 ). 3DE-derived vena contracta area is subject to color Doppler limitations and is also dependent on the proper selection of the systolic frame, which affects measurement accuracy and reproducibility. PISA still requires significant off-line processing and is not practical in a busy clinical setting. Although 3D proximal velocity flow convergence is angle-independent, the lower temporal resolution of 3D color Doppler might affect proper selection of the largest flow convergence region. AROA also requires proper selection of the systolic frame and is limited by the relatively poor temporal resolution of 3DE. 3D mitral inflow and left ventricular outflow tract stroke volume holds great promise, but this method still requires further validation in patients with MR.
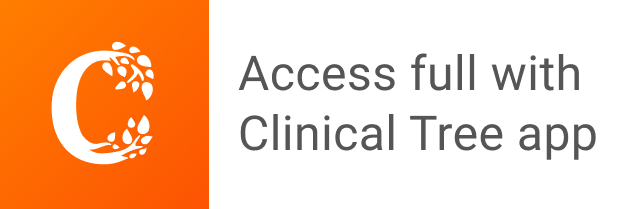