Fig. 14.1
Turning the translational ‘leap’ into a step. The decision to move to a first-in-human study is difficult, but can be considered within the framework outlined in the figure. The case for conducting a first-in-human study is strongest when there is a sound biologic rationale for a new treatment; when the therapy has been successfully tested in appropriate preclinical studies which reliably reflect the human disease; when the potential for adverse events is low; when the probability that the data generated during early phase human studies will shed new light on the pathogenesis of a serious human disease is high; and when there is an important unmet clinical need. It is important to make the distinction between ‘unmet’ and ‘met’ clinical need. In the latter case, while there may be a large burden of disease, effective therapeutic strategies are available and the burden of remaining disease largely relates to ineffective implementation of these strategies (the second translational step). Naturally it is common for not all of these pieces of the puzzle to fall neatly into place, but careful consideration of the weight of evidence along each axis will improve the risk: benefit of a first-in-human study
The burden of lung disease in the twenty-first century—where is the unmet clinical need going to be? Lung disease remains the third most common cause of death globally, behind ischemic heart disease and cerebrovascular disease [56], with most of these respiratory deaths being related to pneumonia. In developing countries tuberculosis remains a very serious problem and smoking-related lung disease (overwhelmingly lung cancer and COPD) causes significant morbidity and mortality worldwide. However a major change is underway in developed nations, with declining cigarette smoking rates, effective antibiotic therapy and population ageing meaning that non-communicable, chronic degenerative diseases will become, beside lung cancer, the major cause of respiratory death in the coming decades [56].
Currently, the most common chronic respiratory disease s in developed nations remain asthma and COPD. In Australia the prevalence of asthma is approximately 10 % and COPD 3 %. Highly effective and safe therapies are already available for asthma so that mortality is now rare, with the major challenges being in achieving a timely and accurate diagnosis, ensuring adherence to treatment to minimise morbidity and mortality, and in identifying the underlying causes to reduce prevalence. COPD on the other hand remains difficult to treat as the structural nature of the disease means that pharmacologic agents are only partially effective, but COPD is completely preventable if cigarette smoking is avoided. Hence COPD-related mortality is declining in developed nations. In contrast, lung cancer remains a leading cause of death, even with declining smoking rates, since a minority of cases are unrelated to cigarette smoking.
If we look ahead to the latter half of the twenty-first century then, the prevalence of COPD and lung cancer will be decreasing but these diseases will remain significant sources of morbidity and mortality. In contrast the prevalence of degenerative and age-related lung diseases will be increasing rapidly without effective treatments to meet this increasing burden. Indeed this change is already underway, with the incidence of IPF, the most common of these diseases, increasing to somewhere (depending on case definition) between 30 and 93/100,000 [57–59] and the annual cumulative prevalence rising rapidly from 202 cases per 100,000 people in 2001 to 494 in 2011 [59]. IPF is a lethal disease with the median survival from diagnosis being 3.5–4 years, even in the modern era [57–59].
Acute lung injury (ALI) is a clinical syndrome of diverse aetiology characterised by widespread pulmonary infiltration and rapidly developing respiratory failure. It is also prominent among lung diseases for its high mortality, lack of effective treatments, and increasing incidence with population ageing. This incidence is now 60–80/100,000 [60], and the mortality rate is 40 % and static [60]. It is likely that ALI will become an increasing problem in developed nations in the coming years. Several pieces of evidence point to a role for MSC treatment in ALI [41, 61–64], and early phase human studies are now underway.
Although therapeutic options for both IPF and ALI remain limited, there has been significant recent progress for patients with IPF, for the first time, confirmation of efficacy of two small molecules in large randomised controlled trials [65, 66]. However, due to a lack of truly effective preventative or therapeutic measures and a rising incidence, in developed nations this century the clinical need will be most pressingly unmet for lung cancer , ALI and IPF. The therapeutic potential of MSCs comes into clear focus when considered from this perspective. It is unlikely that regenerative strategies will play a role in the management of cancer, however they may well go some way to expanding the therapeutic options available for patients with ALI and IPF.
IPF—a degenerative disease in need of a regenerative solution. It is against this backdrop that focus is shifting toward understanding and developing effective therapeutic strategies to manage degenerative lung disease. These non-communicable, non-malignant lung diseases are characterised by failed or ineffective organ repair after injury, with the clinical phenotype (for instance ALI vs. IPF) in large part depending on the nature, severity and acuity of the lung injury as well as host factors such as the effectiveness of repair. In fact IPF is characterised by sudden deteriorations, called exacerbations, which are a form of ALI [67]. In order to appreciate the potential place of future regenerative strategies for human lung disease, it is instructive to take a step back and review recent advances in our understanding of IPF pathogenesis.
Although the moniker ‘idiopathic’ remains appropriate, compelling insight into IPF pathogenesis comes from the relatively rare germline mutations in the human TERT and TERC genes [68]. Together, TERT and TERC form the specialised enzyme telomerase. In stem/progenitor cells the telomerase complex functions to synthesise telomeric DNA and so protect the chromosome ends during cell division. TERT provides reverse transcriptase activity to the complex, and uses TERC, the RNA component of telomerase, as a template. Hence together the TERT and TERC genes maintain telomere length and prevent cellular senescence during recurrent cycles of cellular replication. Telomerase activity is thus a commonly used measure of ‘stemness’, and overexpression of TERT prevents replicative senescence in MSCs and other cells [69]. The most common phenotype in humans with a germline loss-of-function TERT/TERC mutation is a form of pulmonary fibrosis indistinguishable from IPF [70]. Short telomere length, independent of TERT/TERC mutations, is also a strong risk factor for IPF itself, with a clear dose–response relationship between telomere length and survival [71]. These pieces of evidence, along with the epidemiology of IPF (it is overwhelmingly a disease of ageing [58]) indicate that cellular processes which rely on the maintenance of telomere length are fundamental to IPF pathogenesis. A provocative but tantalising conclusion is that IPF results from dysfunction and/or depletion of lung stem/progenitor cell pools over a lifetime. Candidate cells include epithelial progenitors [72], the stromal progenitors which orchestrate epithelial repair , and potentially others, although recent evidence points to depletion of lung resident MSCs in both animal [39] and human [10] lung fibrosis. Repletion of these pools through the delivery of exogenous MSCs or epithelial progenitors [14], or pharmacologic, cell or exosome treatment to improve native adult lung progenitor cell function thus holds promise for the management of IPF [52, 73], ALI [62] and other diseases.
Given this background, it is clear that understanding lung homeostasis and regeneration , and in turn defining the cells which complete these functions, is highly likely to provide new regenerative treatment options. However, currently the ultimate and, at this point, only, regenerative strategy for lung disease is whole organ transplantation, which has now been a viable and evidence-based treatment for selected patients with IPF and other end-stage lung diseases for over three decades. However, lung transplantation necessarily involves the allogeneic replacement of all lung cells, even those with normal function, via a highly invasive operative approach and utilising a very precious and scarce, but also highly immunogenic, resource. More targeted, less invasive and potentially even non- or ‘hypo’-allogeneic approaches to lung regenerative medicine should be possible.
Given the fundamental defects in epithelial repair which underlie these conditions, delivering a cell product like an MSC with the aim of improving repair makes biologic sense, especially since depletion of the MSC pool has now been demonstrated in human IPF [10]. Furthermore, and notwithstanding the limitation of these models, multiple preclinical studies provide robust support for the therapeutic efficacy of MSCs (reviewed by Sinclair et al. [11]) in animal models of lung fibrosis resulting from exposure to bleomycin. Table 14.1 demonstrates the diversity in these studies with respect to cellular source, the use of immunodeficient or immunocompetent animals, and the timing and route of MSC delivery. Most of these studies have utilised allogeneic MSCs which have been isolated from bone marrow using plastic adherence and delivered intravenously or endobronchially. If we return to Fig. 14.1, there appears to be strong evidence for efficacy of MSC treatment in preclinical models of these diseases, but the ability of these models to accurately reflect the corresponding human disease is questionable. This is particularly so for the bleomycin model of pulmonary fibrosis where over the last few decades many compounds have been apparently effective, only to prove ineffective in human trials.
Table 14.1
Preclinical studies of MSCs or MSC-like cells in pulmonary fibrosis
Author | Intervention | Model | Outcome | Engraftment? |
---|---|---|---|---|
Ortiz et al. [74] | Allogeneic 5 × 105 BM-MSC @ 0, 7 days via jugular vein | Mouse bleomycin | ↓ Hydroxyproline—not significant with day 7 infusion | Yes, increased in fibrotic areas |
Cui et al. [75] | BM-MSC @ 1, 7 days via tail vein | Rat bleomycin | ↓ Hydroxyproline and lung fibrotic score—more pronounced with day 1 infusion | Yes |
Zhao et al. [76] | 5 × 106 BM-MSC @ 12 h via tail vein | Rat bleomycin | ↓ Hydroxyproline and pro-fibrotic cytokines | Yes |
Moodley et al. [77] | Xenogeneic umbilical cord-derived MSC 1 × 106 @ 1 day | Mouse bleomycin | ↓ Hydroxyproline, collagen and pro-fibrotic cytokines | Yes, only in fibrotic areas |
Bitencourt et al. [78] | Autologous MSC engraftment encouraged by hyaluronidase | Mouse bleomycin | ↓ Collagen content and fibrotic score | Yes |
Choi et al. [79] | Xenogeneic BM-MSC 2 × 105 IV or microvesicles @ 12 and 14 weeks | Mouse silica | ↓ Collagen content and fibrotic score, more pronounced with MSC | Yes + ATII differentiation |
Yan et al. [51] | Isogeneic BM-MSC 2 × 105 IV @ 0, 60, 120 days | Mouse radiation | ↑ Fibrosis with late delivery | Yes |
Jun et al. [39] | Isogeneic Lung MSC (Hoechst) 2.5 × 105 IV day 0 | Mouse bleomycin | Bleomycin causes lung MSC depletion with repletion attenuating fibrosis | No—?rescue of lung resident MSC |
Although there appears to be a consistent effect of MSCs if delivered soon after the administration of bleomycin, the therapeutic effect diminishes considerably if treatment is delayed until 7 days after administration [51, 74, 75]. This effect highlights a well-known deficiency of the bleomycin model, and is particularly important to recognise since the timing of MSC delivery appears to determine the fate of the engrafting cell, with later delivery favouring the differentiation of MSCs into cells which are pro-fibrotic [51]. The latter possibility remains an ongoing concern for investigators contemplating human IPF trials and will be a key safety outcome. Thus the potential efficacy of MSC-based cell therapy in IPF and ALI remains controversial [80, 81], with the potential for profibrosis being the main drawback. Since MSCs can be driven down a myofibroblastic differentiation pathway given the correct context, these paradoxical findings again highlight the importance of the fundamental work being carried out to discover the secrets of the lung stem cell niche (s), and potentially provide a rationale for delivering therapy earlier in the disease course when epithelial disrepair is at its height, but when extensive fibrosis has not yet ensued. In summary, there is biologic plausibility around MSC treatment for IPF and ALI, there is a large body of preclinical data (admittedly in models which have a questionable relationship to the human disease), there is substantial evidence for safety from human studies for other indications, and there is a large unmet clinical need. From Fig. 14.1, the pieces of the puzzle are in place to proceed to human studies of MSC treatment for IPF and ALI [73], with perhaps a combination of these two conditions, the acute and often lethal, exacerbation of IPF being a prime target.
Aside from lung fibrosis (Table 14.1) and ALI [61, 62], animal studies have provided support for therapeutic efficacy in other lung diseases where the need is currently unmet. This evidence base is well summarised in Sinclair et al. [11] and so will not be repeated here, but is significant for the increasingly common (as survival following extreme preterm birth continues to improve) bronchopulmonary dysplasia [40] and also for pulmonary hypertension [82–84]. With respect to bronchopulmonary dysplasia it is noteworthy that these babies are often left with obstructive lung disease which manifests in adulthood as emphysema [85]. Amongst the preclinical studies in pulmonary hypertension, of particular note are the studies which demonstrated that genetic engineering of MSCs, for instance to overexpress heme oxygenase [83] or prostacyclin [84], conferred enhanced efficacy, perhaps providing a glimpse of the future of the field.
Human Studies of MSC Therapy in Lung Disease
The multifaceted activity of MSC has translated into a large body of clinical trial activity outside the lung , most notably in the treatment of steroid refractory graft versus host disease following allogeneic bone-marrow transplant, but also in other immune-mediated diseases like Crohn’s disease, multiple sclerosis, lupus and in the renal transplant setting [28]. The tissue repair capability of MSCs is being investigated in clinical trials for cardiac repair, bone disorders (osteogenesis imperfecta), bone fracture and following meniscectomy. As a result, many thousands of human subjects have received intravenous MSC therapy with very few adverse effects [86], providing key safety data for moving to clinical trials in lung disease. While relatively few human trials are underway for patients with lung disease, and while even fewer have been published, the diseases which have been targeted reflect MSC biology, the strength of the preclinical data, and the seriousness and lack of availability of alternate treatments for the target disease.
Chronic obstructive pulmonary disease: Weiss et al. conducted what remains the largest trial in humans with lung disease using allogeneic bone-marrow-derived MSCs [53]. They randomised 62 patients with COPD to receive 4 monthly intravenous infusions of either MSCs (100 × 106 cells) or vehicle control in a double-blind manner. Patients were followed for 2 years. There were no infusional toxicities and no treatment-related deaths or serious adverse events. Although MSC treatment was not associated with any improvement in the efficacy measures (lung function , walk distance, or dyspnea score), this study does provide excellent safety data in humans with moderate-to-severe lung disease [53]. Another study targeting emphysema is currently listed as recruiting (NCT01849159, www.clinicaltrials.gov, accessed 20th Feb 2015).
Obliterative bronchiolitis: Recruitment to a phase I trial of MSC therapy for post-transplant obliterative bronchiolitis has recently been completed (http://clinicaltrials.gov/ct2/show/NCT01175655). In this study ten patients with moderate or severe chronic lung allograft dysfunction received allogeneic bone-marrow-derived MSCs (2 × 106 cells/kg twice weekly for 2 weeks) and will be followed for 1 year. MSC therapy was feasible and appeared well tolerated in the short-term, with long-term results awaited [32]. A similar Phase 1 study has recently commenced recruitment (NCT02181712) in the United States. A Phase 2 study is now being planned in Australia.
IPF: The short-term safety of MSC treatment in IPF was recently confirmed in a Phase 1 study of intravenous, allogeneic, placenta-derived MSC in moderate-severely affected patients [52]. In this study, eight patients were treated with 1–2 × 106 MSCs/kg and were followed for 6 months. There was no evidence of acute hemodynamic or gas exchange compromise and no evidence of worsening fibrosis [52]. In the only other published human study, 14 patients with IPF received 0.5 × 106/kg autologous adipose-derived stromal cells endobronchially. No adverse effects of this treatment were seen. Two other clinical trials are ongoing in IPF. A US trial with very similar design to the study by Chambers et al. but involving intravenous delivery of allogeneic bone-marrow-derived MSCs, has almost completed recruitment (NCT02013700, M. Glassberg personal communication) while the other trial (NCT01919827) involves the non-randomised endobronchial delivery of allogeneic adipose-derived MSCs.
ALI: No studies have been published for MSC treatment of ALI, but there are currently two randomised clinical trials and one non-randomised trial underway. The first (NCT01775774) is a dose-escalation (1 × 106, 5 × 106, and 10 × 106 cells/kg) study delivering allogeneic bone-marrow-derived MSCs to three cohorts of patients (n = 3/cohort). The other randomised, double-blind, placebo-controlled trial (NCT01902082) delivers allogeneic adipose-derived MSCs. The inclusion criteria are similar for these two studies. In the third non-randomised study, patients with viral infection-induced and extra-corporeal membrane oxygenation-dependent ALI will receive open-label allogeneic bone-marrow-derived MSCs (NCT02215811). In all three studies MSCs are delivered intravenously.
Other disease targets: The only other clinical trials of MSC therapy for lung disease listed as active at www.clinicaltrials.gov are in bronchopulmonary dysplasia, where a Phase 1 study has recently reported promising results [87]; in pulmonary hypertension (NCT01795950) where a Phase 1 study involves three dosing cohorts of 0.5–2 × 106 placenta-derived MSCs/kg delivered intravenously; and in radiation-induced lung fibrosis (NCT02277145).
Conclusions
Together, this preclinical and clinical trial activity showcases the diversity and potential of MSCs in regenerative medicine . MSCs have been shown to be much more than a cellular population with immune-suppressive activity and multipotent capacity. MSCs can also promote tissue repair by regulating the functionality of tissue stem cell pools and rescuing aged, senescent or damaged tissue. While a number of lung diseases, most notably asthma, are now able to be relatively safely and effectively treated due to significant improvements in available pharmacologics, substantial therapeutic gaps remain, particularly for degenerative and fibrotic lung diseases. It is pleasing to think that a deeper understanding of the role of tissue-resident stem cells in the maintenance of lung health, and hence the development of pharmacologic and/or cell therapies aimed at restoring that health, may one day fill these gaps. However in order for this promise to be achieved safely, and in order to avoid a repetition of the problematic headlong introduction of gene therapies in large-scale clinical trials [88], a deeper understanding of basic MSC biology is needed, alongside the careful conduct of early phase human trials. The elucidation of the interrelationships between epithelial and MSC hierarchies; the role of MSCs in the construction of lung stem cell niches; and the dynamics of the cellular interactions within these niches are key to achieving this objective.
References
1.
Prockop DJ, Prockop SE, Bertoncello I (2014) Are clinical trials with mesenchymal stem/progenitor cells too far ahead of the science? Lessons from experimental hematology. Stem Cells 32(12):3055–3061PubMedCentralCrossRefPubMed
2.
Friedenstein AJ, Chailakhjan RK, Lalykina KS (1970) The development of fibroblast colonies in monolayer cultures of guinea-pig bone marrow and spleen cells. Cell Tissue Kinet 3(4):393–403PubMed
3.
Friedenstein AJ, Piatetzky-Shapiro II, Petrakova KV (1966) Osteogenesis in transplants of bone marrow cells. J Embryol Exp Morphol 16(3):381–390PubMed
4.
Lama VN, Smith L, Badri L, Flint A, Andrei AC, Murray S et al (2007) Evidence for tissue-resident mesenchymal stem cells in human adult lung from studies of transplanted allografts. J Clin Invest 117(4):989–996PubMedCentralCrossRefPubMed
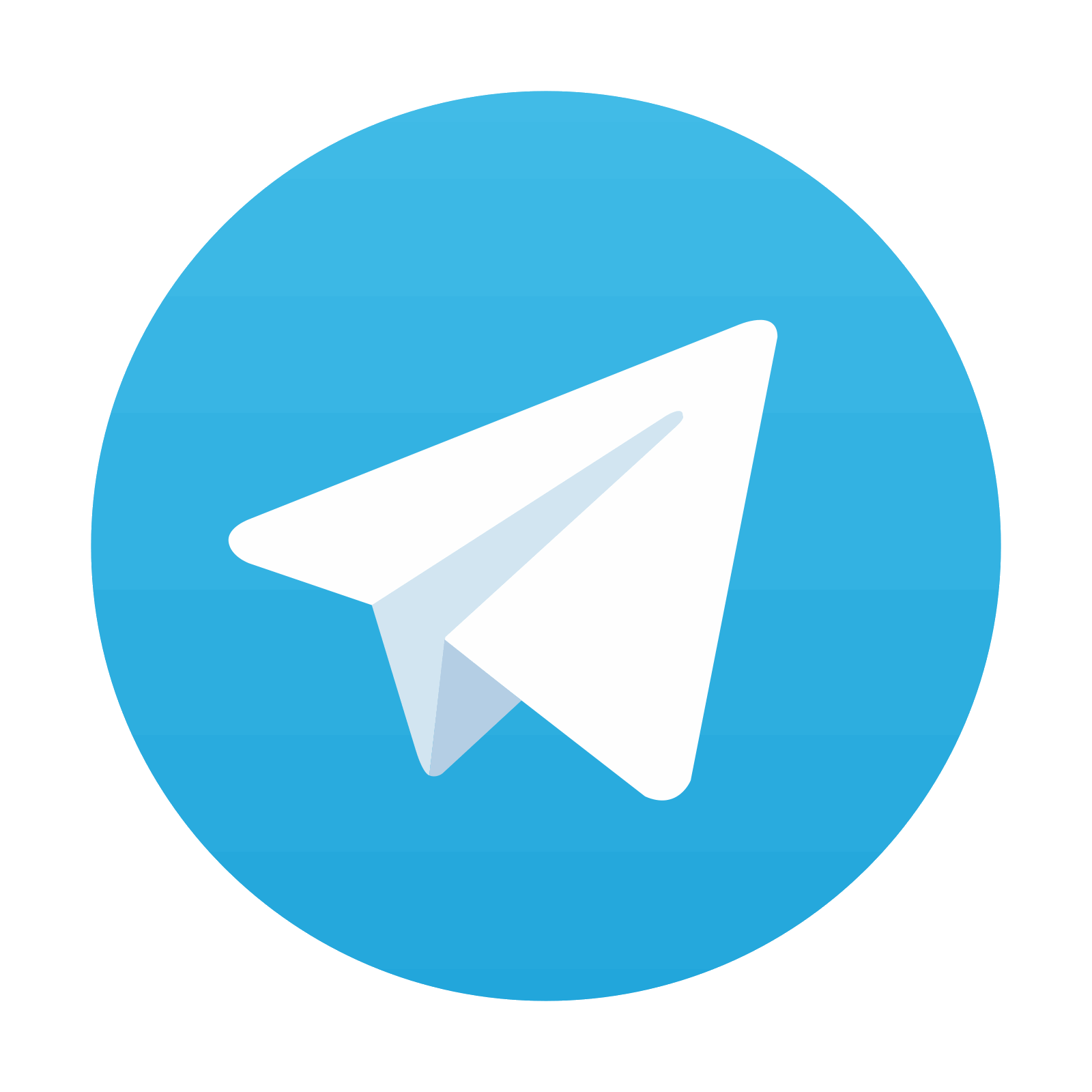
Stay updated, free articles. Join our Telegram channel
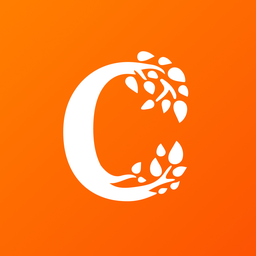
Full access? Get Clinical Tree
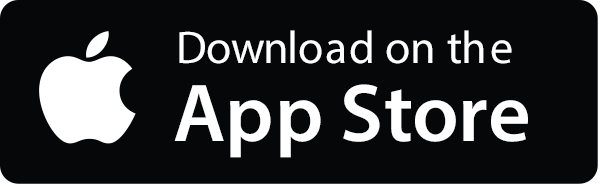
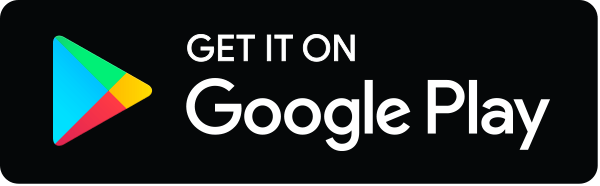