Mechanical Ventilation of the Surgical Patient
James E. Lynch
Joseph B. Zwischenberger
Although there are references to artificial respiration from biblical times (Second Kings 4:32–35), the practical application of mechanical ventilation in patients with respiratory distress became available only in the last half of the twentieth century and has been revolutionized within the last 10 to 15 years. Postoperative support of the surgical patient is frequently uncomplicated and time-limited, as most patients are extubated immediately following surgery. However even with the latest ventilatory techniques, some patients will suffer barotrauma, volutrauma, biotrauma, or ventilator-acquired pneumonia (VAP), or they may develop acute respiratory distress syndrome.
Indications for Mechanical Ventilation
Little research and no clinical trials have been performed on the indications for mechanical ventilation. Many trials and much literature exist regarding the discontinuation of mechanical ventilation. Some have attempted to use these data to extrapolate criteria for initiating mechanical ventilation, such as developing threshold values for tidal volume, minute ventilation or PaCO2. However, these objective criteria are often ignored, because the need for mechanical ventilation usually represents a deterioration of both biochemical and physical measurements. No one number or value is likely to be sensitive or specific enough to guide a clinician as to the need for mechanical ventilation. Indications for mechanical ventilation are best derived from careful bedside observation of the patient. The primary indications for mechanical ventilation are either inadequate ventilation, an unsustainable level of ventilatory demand, or refractory hypoxemia. Simply stated, the patient exhibits respiratory distress.
Postoperative Support
The need for mechanical ventilation in the postsurgical period can stem from a number of causes. Sometimes the need for mechanical ventilation is expected as part of the recovery process from a major operation. Ventilatory support may also be needed for an intraoperative or postoperative complication or change in patient condition. Major causes include:
Inadequate alveolar ventilation
Diminished or unstable ventilatory drive
Severe hypoxemia
Inadequate lung expansion
Excessive work of breathing
Underlying disease and general physical health of the patient must be considered. Once it is determined that a patient will require mechanical ventilation, the mode of delivery (noninvasive positive pressure ventilation [NPPV] versus conventional mechanical ventilation), initial settings, and adjunctive treatments must be considered.
Siafakas et al.144 noted little change in the postoperative minute ventilation while there was significant alteration of many other measures of respiratory mechanics, especially following thoracic or upper abdominal surgery.86 Changes include reduction in the vital capacity (VC), tidal volume (VT), and functional residual capacity (FRC), leading to atelectasis which worsens ventilation/perfusion matching. An increase in the respiratory rate (RR) therefore compensates to maintain minute ventilation. Hypoventilation resulting from pain or sedation can lead to primary ventilatory failure from acute CO2 retention or an inadequate level of arterial oxygenation (oxygenation failure). These altered mechanics with concurrent atelectasis in postoperative patients increases the risk of infection.
Postoperative respiratory failure is defined as the need for intubation and mechanical ventilation in the 48 hours following an operation.10 Several factors must be considered in evaluating the patient with impending respiratory failure no matter the etiology. Relevant questions one should ask include:
Can this patient adequately protect the airway?
Would this patient benefit from a trial of noninvasive positive pressure ventilation?
Is the current respiratory pattern sustainable?
Is this patient getting better or worse?
A negative response to these questions will guide the clinician in the use of mechanical ventilation.
Inadequate Alveolar Ventilation
Although PaCO2 is a direct reflection of alveolar ventilation, pH may be a more useful monitoring tool. In a patient with long-standing lung disease such as chronic obstructive pulmonary disease (COPD), an elevated PaCO2 with a normal pH is not an abnormal finding. More troublesome than simply an elevated PaCO2 is an elevated PaCO2 with a decreased pH (<7.30), which is indicative of acute respiratory failure. Worsening respiratory acidosis is an indication for ventilatory assistance. NPPV has gained popularity in the last few years as the initial step toward respiratory support.103,119 Most patients not requiring emergent intubation should be given a short trial (30–60 minutes) of NPPV.72 However, even with predictors and a growing experience in NPPV, failure rates remain high9,47 and necessitate intubation in 30% of patients.
The Immediate Postoperative Period
The clinical signs of impending respiratory muscle fatigue must be recognized and corrective measures taken before these signs are reflected in abnormal arterial blood gas values. Such recognition requires bedside assessment of the patient rather than laboratory data. Mental acuity and awareness are the most important indicators. Postoperative respiratory failure can occur in one of three situations: ventilatory failure due to inadequate reversal of anesthesia or narcotic overdosage, perioperative aspiration, or airway obstruction. Adequate reversal of narcotics with naloxone is frequently all that is required for the patient with drug-induced hypoventilation and acute changes in pH and PaCO2. In the case of inadequate reversal, if mechanical ventilation is required, the duration is frequently short.
Perioperative aspiration can be more problematic, resulting in a chemical pneumonitis or Mendelson’s syndrome.108 Aspiration often results from inadequate upper airway defense due to anesthesia. Patients who have aspirated often will present with an abrupt onset of tachypnea and tachycardia; symptoms may include bronchospasm, fever, or cyanosis.174 These patients may require mechanical ventilation, and their course can become complicated. In the patient with suspected aspiration and acute changes in PaCO2, reintubation and a chest radiograph are indicated. Changes in the chest radiograph are usually noted within 2 hours. The mainstay of treatment is pulmonary support. Empiric treatment with corticosteroids159 and antibiotics105 is controversial and discussed in more detail elsewhere.
Postoperative airway obstruction stems from a number of etiologies including laryngeal edema, laryngospasm, and obstruction. Laryngeal edema resulting in stridor constitutes a medical emergency; such patients should be evaluated by a physician with experience in managing difficult airways.
Diminished or Unstable Ventilatory Drive
Frequently the etiology of a diminished respiratory drive is metabolic, manifested by either a decreased respiratory rate or periods of apnea. Without immediate reversal, mechanical ventilation will be required. Other causes of unstable ventilatory drive include closed head injury, drug overdose, or massive cerebrovascular accident. This setting is not appropriate for NPPV; these patients should be treated primarily with intubation and mechanical ventilation.
Severe Hypoxemia
Mild to moderate hypoxia can frequently be managed with the administration of supplemental oxygen. The cause of the hypoxia should always be assessed. Classically, alterations in oxygenation can be classified as either alveolar hypoventilation, oxygen diffusion deficit, intrapulmonary shunt, or a ventilation/perfusion mismatch.132 These changes in the lung can be due to a number of factors both present before and resulting from surgical procedures. To understand how to best improve alterations of oxygenation one must appreciate the physiologic basis of normal alveolar oxygen transfer.
Oxygen tension is maintained in humans as a balance between oxygen delivery and oxygen consumption. Interestingly, the relationship between delivery and consumption is not linear; instead, there is a “plateau phase” (Fig. 42-1) in which oxygen consumption is maintained at a relatively constant rate until a critical oxygen delivery ([D with dot above]o2) value is reached at which point the body shifts from oxidative phosphorylation to anaerobic
glycolysis. At this point, lactate, a by-product of anaerobic glycolysis, begins to rise.35 This plateau phase is possible because of an increase in oxygen extraction from the blood. Oxygen extraction is the ratio of arterial oxygen content to that of mixed venous blood. As extraction increases, mixed venous saturation will decrease, while arterial saturation and oxygen consumption levels remain fairly constant. This relationship holds true until a certain threshold is reached. This critical [D with dot above]o2 level has been found in a small series of dying patients to be 4.5 mL/kg per minute with an oxygen extraction ratio of 60%.133 Unfortunately, physiologic regulation becomes less efficient in critically ill patients. The delivery/consumption relationship depends on the ability of the body to react to falling levels of [D with dot above]o2 through several mechanisms. Since delivery is a combination of oxygen content and cardiac output, the cardiac response to falling levels of oxygen content is vital. Without an intact cardiac response, the critical [D with dot above]o2 level can be altered and patients can exhibit signs of tissue hypoxia at a [D with dot above]o2 level higher than what would be expected in the nonpathologic state. The ability of the body to redistribute blood flow in response to decreased oxygen delivery is also affected by severe disease states such as sepsis and acute respiratory distress syndrome (ARDS). Likewise, the ability of the body to increase oxygen extraction is frequently less in several pathologic conditions.
glycolysis. At this point, lactate, a by-product of anaerobic glycolysis, begins to rise.35 This plateau phase is possible because of an increase in oxygen extraction from the blood. Oxygen extraction is the ratio of arterial oxygen content to that of mixed venous blood. As extraction increases, mixed venous saturation will decrease, while arterial saturation and oxygen consumption levels remain fairly constant. This relationship holds true until a certain threshold is reached. This critical [D with dot above]o2 level has been found in a small series of dying patients to be 4.5 mL/kg per minute with an oxygen extraction ratio of 60%.133 Unfortunately, physiologic regulation becomes less efficient in critically ill patients. The delivery/consumption relationship depends on the ability of the body to react to falling levels of [D with dot above]o2 through several mechanisms. Since delivery is a combination of oxygen content and cardiac output, the cardiac response to falling levels of oxygen content is vital. Without an intact cardiac response, the critical [D with dot above]o2 level can be altered and patients can exhibit signs of tissue hypoxia at a [D with dot above]o2 level higher than what would be expected in the nonpathologic state. The ability of the body to redistribute blood flow in response to decreased oxygen delivery is also affected by severe disease states such as sepsis and acute respiratory distress syndrome (ARDS). Likewise, the ability of the body to increase oxygen extraction is frequently less in several pathologic conditions.
The importance of the delivery/consumption relationship has led to the development of several measurement techniques to allow for determination of adequate oxygen delivery ([D with dot above]o2) relative to oxygen consumption ([V with dot above]o2) in severely ill patients. While the details of these measurement techniques can be found elsewhere, an important consideration in selecting a measurement system includes avoidance of technology where [D with dot above]o2 and [V with dot above]o2 are calculated from common variables (mathematical coupling), as this can lead to serious alterations in the measured versus actual values. Current recommendations center around the use of clinical assessment of oxygenation status to guide therapy in critically ill patients on mechanical ventilation. Hypotension, tachycardia, and poor capillary refill are all signs of inadequate oxygen delivery. In the absence of other causes, these symptoms should point to the need to increase oxygen delivery by at least 25%.136
The effects of mechanical ventilation on oxygenation are numerous. Although the ventilator is primarily simply a way of improving gas exchange through the expansion of the functional residual capacity and the delivery of increased FIO2, an important secondary effect is the redistribution of blood flow away from the respiratory muscles to other vital organs.97 In studies of cardiogenic shock in spontaneously breathing dogs, the percentage of cardiac output utilized by the respiratory muscles can be as high as 21% (normal is 1%–3%). Upon initiation of mechanical ventilation, this number returned to normal.171 In humans, intubation and mechanical ventilation have been shown to decrease global oxygen consumption by 20%.97 This inverse of decreasing oxygen consumption in patients on mechanical ventilation applies to patients upon withdrawal of mechanical ventilation and should be kept in mind when patients are weaned from mechanical ventilation in the early perioperative period.123
Recognition of hypoxemia can be difficult. Cyanosis is considered to be the hallmark of hypoxemia; however, many variables affect the degree to which cyanosis can be detected. Thickness and opacity of the skin as well as the skill of the observer all affect the ability to detect cyanosis. Central cyanosis is believed to result from 5 g/dL of deoxygenated blood in the capillaries, but this has been difficult to substantiate.34 Interrater agreement in the observation of cyanosis is reported at 53% to 69%, while 45% of patients observed to have cyanosis of the tongue had normal SaO2.107 Therefore cyanosis as the lone marker of hypoxia is inadequate.
Oxygenation in Different Disease States
As stated earlier, derangements in gas exchange stem from four basic physiologic disorders: alveolar hypoventilation, oxygen diffusion deficit, intrapulmonary shunt, or a ventilation/perfusion mismatch. During mechanical ventilation, the two main determinants of arterial oxygen tension are positive end-expiratory pressure (PEEP) and FIO2. The extent to which PEEP influences PaO2 is controversial and depends largely on the disease process being studied. In acute respiratory distress syndrome (ARDS) or acute lung injury (ALI), the use of moderate levels of PEEP reduces shunt and physiologic dead space. Postoperative hypoventilation frequently results from drug depression of the respiratory pattern generator in the medulla. The extent to which CO2 is elevated is proportional to the depression observed in PaO2.
Impairment of diffusion, while usually not an acute problem, can be aggravated by small decrements in respiratory function that frequently accompany thoracic surgery. Common diseases that affect the diffusion of gas across the alveolar membrane include sarcoidosis, asbestosis, and interstitial fibrosis. Because of the near linearity of the CO2 diffusion curve (CO2 diffuses 20 times faster than O2) hypercapnia almost never results from a diffusion problem.
Shunts can be anatomic or physiologic. Anatomic shunts result when blood passes from the right side of the heart to the left side without going through the lungs. Anatomic shunts are frequently the result of congenital heart defects, but they can develop following a myocardial infarction. Physiologic shunts are the result of blood flow through unventilated alveoli. Frequent causes of physiologic shunts include pneumonia, atelectasis, pulmonary edema, and pneumothorax. Since shunts are the result of blood passing by a collapsed alveolus, they are frequently refractory to supplemental oxygen.
Inadequate Lung Expansion
Inadequate lung expansion can be present even in a patient with normal blood gases. Ineffective, shallow breathing in postoperative patients is common and can be the result of thoracic or abdominal trauma or surgery, morbid obesity, a restrictive pulmonary disease, or underlying neuromuscular disease. The commonly observed pattern usually manifests in a rapid shallow breathing pattern with a respiratory rate ≥30 and small tidal volumes. Tobin and Yang178 observed that patients who breathed in a rapid shallow pattern were less likely to wean from mechanical ventilation. This failure to wean was due in part to inadequate lung expansion.
Atelectasis is the most common pulmonary postoperative complication, occurring in 90% of patients undergoing general anesthesia.56 In patients undergoing cardiac or thoracic operations, ≥50% of the lung is often collapsed several hours postoperatively.152 Proposed mechanisms for the development of intraoperative atelectasis have included decreased muscle
tone, reduction in functional residual capacity from cephalad displacement of the diaphragm, direct compression of the lung tissue, surfactant dysfunction, and absorption atelectasis from high FIO2.59 Postoperative pain and diaphragmatic dysfunction leads to decreased vital capacity and functional residual capacity.83 Mucociliary clearance is impaired while narcotics and poor pain control suppress deep breathing and normal cough reflexes, leading to retained secretions.121 Bonde et al.,19 in a prospective study, found that pulmonary complications following thoracic surgery occurred in 30% of patients. By using multivariate analysis, they found that independent risk factors included current smoking, ischemic heart disease, and absence or failure of regional analgesia.
tone, reduction in functional residual capacity from cephalad displacement of the diaphragm, direct compression of the lung tissue, surfactant dysfunction, and absorption atelectasis from high FIO2.59 Postoperative pain and diaphragmatic dysfunction leads to decreased vital capacity and functional residual capacity.83 Mucociliary clearance is impaired while narcotics and poor pain control suppress deep breathing and normal cough reflexes, leading to retained secretions.121 Bonde et al.,19 in a prospective study, found that pulmonary complications following thoracic surgery occurred in 30% of patients. By using multivariate analysis, they found that independent risk factors included current smoking, ischemic heart disease, and absence or failure of regional analgesia.
Excessive Work of Breathing
Many conditions can lead to an increase in the work of breathing, including asthma, COPD, cardiogenic pulmonary edema, and acute respiratory distress syndrome (ARDS). Increased work of breathing manifests in both physical and biochemical markers. Signs of increased work of breathing include increased use of accessory muscles, paradoxical abdominal movement, nasal flaring, and tachypnea.176,177 Tachypnea has been called “the near universal sign of accompanying respiratory distress.”84 Obtaining an accurate respiratory rate requires a longer measure than the 15 seconds normally afforded. Studies have shown variation of 20% from the actual value in 40% of nurses tested.78
Although tachypnea almost always accompanies respiratory distress, rapid respiratory rates are not necessarily a sign of impending respiratory failure or the need for mechanical ventilation. In fact, in a normal person, doubling of the respiratory rate and minute ventilation can be sustained indefinitely. However, in the setting of acute illness or underlying pulmonary disease, increased respiratory rates and minute ventilation in order to maintain a normal PaCO2 can be a sign of impending collapse. In disease states, altered respiratory mechanics—such as increased airway resistance or reduced lung compliance—can result in markedly increased work of breathing for a given level of ventilation. Therefore increases in ventilatory demand can result in an unsustainable workload, leading to respiratory muscle failure.
Hypercapnia ensues as a patient’s respiratory musculature fatigues and ventilation begins to fall. Prior to catastrophic decompensation, carbon dioxide levels are often “normal,” but only because elevated minute ventilation compensates for reduction in gas-exchange efficiency (e.g., increased dead space). Therefore the level of PCO2 is relatively insensitive to respiratory failure.
Options in Ventilatory Support
The goal of any ventilatory assist mode is to allow for normal or near normal gas exchange while the underlying pathophysiology that resulted in the need for mechanical ventilation resolves. There are numerous modes and modalities for the delivery of mechanical ventilation, with more being developed and tested even at the time of publication of this text. The following pages cover some of the major modes and more widely utilized methods for ventilation.
Noninvasive Ventilation
The recent literature is filled with reports on the use of noninvasive positive-pressure ventilation (NIPPV) in various populations (Table 42-1). Currently there is evidence for the use of NIPPV in severe exacerbations of COPD,33,34,35,36 cardiogenic pulmonary edema,112 and immunocompromise with respiratory failure.8,63 The use of NIPPV as an adjunct to weaning or for post- extubation respiratory distress is discussed later in this chapter. The concept of NIPPV in the postoperative thoracic surgery patient has also been specifically studied. In coronary artery bypass (CABG) patients, NIPPV (specifically bilevel support) improved measurable lung mechanics and measures of oxygenation over chest physiotherapy and continuous positive-pressure ventilation (CPAP) alone.104 NIPPV was also shown to be superior to oxygen therapy alone in patients following lung resection.3 In a recent study by Auriant et al.,11a 48 patients were randomized to conventional therapy or NIPPV when they developed respiratory insufficiency after lung resection. NIPPV reduced the need for intubation (21% versus 50%) and reduced mortality (13% versus 38%). This evidence supports the use of NIPPV in the postoperative thoracic surgery patient prior to intubation.
In spite of the mounting evidence in favor of NIPPV, some doubt still remains regarding its use in patients with respiratory distress. A recent study showed that the delay caused by a trial of NIPPV prior to intubation led to an increase in mortality in that group.50 Also, the methodology of most trials limits interpretation. For example, in most trials, patients who are started on NIPPV and not intubated are considered to have avoided intubation. In reality, this determination is difficult to make because the threshold for intubation is different from that of NIPPV. Not every patient who is placed on NIPPV is in need of intubation. These limitations, coupled with the fact that there are no universal criteria for intubation, make interpretation of the NIPPV literature difficult. Caution must be exercised in the application of NIPPV and care taken to ensure that an appropriate level of
monitoring is available, because despite favorable reports, one-third of patients on NIPPV still require intubation.
monitoring is available, because despite favorable reports, one-third of patients on NIPPV still require intubation.
Table 42-1 Characteristics of Patients Successfully Treated with Noninvasive Positive Pressure Ventilation | ||||||||||
---|---|---|---|---|---|---|---|---|---|---|
|
Patient–ventilator interaction in NIPPV is as much an art as science and requires constant adjustment. Key to the successful application of NIPPV is selection of the equipment, including selection of the mask, ventilator, and initial settings. A growing number of masks and NIPPV interface devices have become available. Detailed description and the positives and negatives of each device are beyond the scope of this chapter.143 Basic designs of interface devices include full face mask, nasal mask, and helmet design. A study by Kwok et al.80 demonstrated no difference in improvement of symptoms between the full face mask and the nasal mask. Initial tolerance was higher in the nasal mask group (88% versus 66%). The helmet design, which fits over the entire head and seals around the shoulders, has shown improved tolerance when compared with the full face or nasal masks.45,46,47 Of some concern is the need for high flow rates to limit rebreathing, leading to a very noisy environment inside of the helmet.30 Given all of the available choices, patient comfort and fit are the most important factors in selecting an interface device.
The ventilator options for NIPPV have steadily grown over the last 10 years. Many NIPPV ventilators still suffer from the lack of alarms and monitoring devices, which preclude their use outside of the ICU. NIPPV ventilators usually consist of a single supply hose for inhalation and exhalation, leading to some rebreathing at low levels of expiratory pressure. Use of a minimum expiratory pressure of at least 4 cm H2O can help to minimize the amount of rebreathing.51 Although rebreathing can also be minimized with the use of one-way exhalation valves, these valves have been shown to increase work of breathing.51,92 In-mask exhalation valves have shown better performance without increases in work of breathing.137,142
Some ventilators designed for invasive ventilation now come with noninvasive modes. These allow for adjusted sensitivity and leak compensation necessary for NIPPV. These features overcome many problems that have made the use of traditional ventilators problematic. In comparing the traditional ventilator with a ventilator designed specifically for NIPPV,118 both perform equally well in improving gas exchange and reducing the work of breathing when expiratory pressures are ≤5 cm H2O. While there has been no outcome study that compares dedicated NIPPV ventilators with standard ventilators, no difference in outcome is apparent between trials that have used one or the other ventilator. Consideration of which ventilator is right in each situation should be based on availability, level of monitoring, cost of disposables, and operator expertise.
Some authors have argued that, in using bilevel support, the inspiratory positive airway pressure (IPAP) should be started high (>20 cm H2O) and titrated down as the patient tolerates to allow for immediate relief of dyspnea,34 while others have argued for a lower initial inspiratory pressure (<10 cm H2O), which is slowly increased until the respiratory distress is alleviated.76 No studies to date have shown any one method of setting the IPAP level to be superior to another. Setting the level of expiratory positive airway pressure (EPAP, sometimes referred to as CPAP) is usually determined by oxygen saturation/PaO2; however, most investigators agree that high levels of EPAP are not well tolerated and therefore not recommended.
Rates can be set on some of the newer NIPPV ventilators and all traditional ventilators. Rates were initially used in patients with neuromuscular or central apnea. However, more clinicians have started to place patients on rates on NIPPV to improve gas exchange.
Invasive Mechanical Ventilation
Systematic comparison of one ventilator with another or one mode with another in randomized trials is rare; generally selection of a ventilator or mode depends on what is available.
Selecting a Mode of Mechanical Ventilation
Volume Versus Pressure Modes
Pressure-targeted modes ensure pressure limits at the expense of variability in tidal volume, while volume-targeted modes guarantee flow and consequently tidal volume within a preset inspiratory time at the expense of airway pressure variability. With pressure-targeted modes, the targeted inspiratory pressure and inspiratory time must be selected with consideration of the desired tidal volume and then constantly monitored, because changes in lung mechanics will lead to changes in tidal volume. If volume-controlled ventilation (VCV) is used, a tidal volume and flow delivery pattern (waveform and peak flow) is selected and peak airway pressures must be constantly monitored because changes in lung mechanics will lead to changes in peak airway pressure. Volume-targeted modes provide a preset volume unless a specified pressure limit is exceeded. Pierson122 and Hess and Kacmarek70 showed that major advantages with volume-targeted ventilation include the capacity to deliver a predictable VT, the flexibility of flow and volume adjustments, and the guarantee of a preset minute ventilation. In volume-targeted ventilation, alterations in airway resistance or lung compliance will alter airway pressures but will not affect minute ventilation. The advantages and disadvantages of volume versus pressure modes are well known; in a recent survey, the use of pressure-control ventilation had not increased,48 suggesting that individual physician preference might be driving the choice of ventilator mode. The current literature consists of several studies that have shown advantages to volume modes, including lower mean airway pressures,9,37 improved shunt,185 and several that have shown advantages to pressure ventilation,1,111 including a recent study showing improved gas distribution at end expiration.124
Volume-Targeted Modes
Assist-Control Ventilation (ACV)
ACV is a volume-control mode that delivers a preset tidal volume on every inspiratory effort or a set baseline respiratory rate (Fig. 42-2). It is the most common mode of ventilation currently used in ICUs.49 In ACV, the “mandatory” rate is the number of breaths that will be delivered if no patient effort is sensed by the ventilator. In addition to the mandatory rate, any inspiratory effort will result in a delivered breath at the preset tidal volume and flow rate as the mandatory breath. This results in a guaranteed minimum minute ventilation. In many ventilators peak inspiratory flow rates can be set, while in others inspiratory time is set
in ACV. Either way, volume is delivered based on the product of the inspiratory time and the peak flow rate and can be delivered in either a square, sinusoidal, or ascending/descending ramp wave pattern.
in ACV. Either way, volume is delivered based on the product of the inspiratory time and the peak flow rate and can be delivered in either a square, sinusoidal, or ascending/descending ramp wave pattern.
Spontaneous breaths are sensed by the ventilator either by a pressure trigger or a flow trigger. Pressure triggers utilize a microprocessor to sense a drop in pressure from the baseline pressure by a set amount (usually 2–4 cm H2O) to open the demand valve and deliver a breath. Flow triggering has been shown to be superior to pressure triggering in pressure support ventilation but not in the ACV mode.64,65,66 It relies on small inspiratory efforts that are sensed as inspiratory efforts and trigger a breath from the ventilator. Setting of the trigger sensitivity requires close observation of the patient, because if the sensitivity is set incorrectly, it can result in missed inspiratory efforts and increased work of breathing102 or in autocycling of the venti- lator.
ACV is utilized when complete unloading of the respiratory muscles is the goal during severely increased work of breathing or derangements of gas exchange.96 ACV allows for a minimum minute ventilation and for easy measurement of most respiratory parameters including intrinsic PEEP, compliance, resistance, and work of breathing. Limitations of ACV include the inability to tailor a specific ventilator setting to patient demand. On each inspiratory effort, inspiratory time, flow rate and tidal volume are not under patient control. Depending on the settings, the ventilator might have a shorter cycle time than the patient’s respiratory pattern generator in the pons, resulting in premature termination of the breath and patient dysynchrony. ACV is also not very responsive to changes in lung compliance. This lack of synchrony can result in the patient “fighting the ventilator.” Unfortunately, a common response is to increase sedation, when the better alternative would be to choose another mode of ventilation.
Intermittent Mandatory Ventilation (IMV)
IMV was first described as a ventilatory mode in 195517; it gained wide acceptance as a means of weaning and as support for acute respiratory failure in the 1970s.70,71,72 IMV allows for a set “mandatory” rate and, unlike ACV, in which breaths between mandatory breaths triggered the preset tidal volume and flow rate, in IMV the patient is allowed “spontaneous” breaths. These spontaneous breaths could be supplemented with airway pressure, allowing for a more patient-tailored breath. The first IMV ventilators delivered a mandatory breath regardless of whether the patient was in the middle of a spontaneous breath. This problem was addressed with the advent of synchronized IMV (SIMV). In SIMV, the ventilator utilizes a demand valve to synchronize the mandatory breaths with the patient’s inspiratory effort. This is currently the standard in most ICUs around the world. In this section, IMV and SIMV are considered to be the same.
Early thinking behind the development of IMV ventilation was that ACV caused downregulation of diaphragmatic muscle activity and led to muscle wasting.39 IMV with mandatory and spontaneous breathing would allow for periods of rest and periods of activity of the diaphragm. By slowly decreasing the mandatory rate, the percent of work the diaphragm was performing could slowly be increased. However, this theory has been disproved by several later studies.73,74,75,76 Even at varying levels of support, no significant difference in neuromuscular output from the diaphragm could be detected between spontaneous and mandatory breaths.65 The idea that breath-to-breath adaptation is based on a level of assistance from the ventilator does not appear to be accurate.
At all but the highest levels of support in IMV, the work of breathing (WOB) is increased when compared with a comparable level of support in ACV.101 In addition to increased WOB, during IMV mode, respiratory muscle effort breath to breath did not vary. IMV was also seen as a more “patient friendly” mode because it allowed the patient to control flow rate, tidal volume, and inspiratory time on spontaneous breaths. No studies, however, have shown a benefit in terms of total amount of sedation in comparisons of CMV with IMV.141 Some have advocated the use of IMV to reduce the respiratory alkalosis that can be seen in patients on CMV. While IMV has been shown to reduce the amount of alkalosis, this comes at the expense of increased WOB.55,101
The use of IMV in the weaning of patients from mechanical ventilation gained popularity in the late 1970s and early 1980s. The gradual reduction in IMV rate while allowing more spontaneous breathing by the patient was thought to be beneficial. Two large randomized trials that examined weaning methods in patients on mechanical ventilation concluded that SIMV resulted in longer weaning times than T-piece weans or weaning utilizing pressure support ventilation.21,46
IMV remains the most popular ventilator mode among North American physicians.49 However, it does not shorten weaning
times, allow diaphragmatic rest, or improve gas exchange compared with ACV. While few studies show that IMV is harmful (except in weaning, where it prolongs the need for mechanical ventilation), it is not the panacea that was envisioned when it was created.
times, allow diaphragmatic rest, or improve gas exchange compared with ACV. While few studies show that IMV is harmful (except in weaning, where it prolongs the need for mechanical ventilation), it is not the panacea that was envisioned when it was created.
Pressure-Targeted Modes
Pressure-Control Ventilation (PCV)
PCV utilizes a specific targeted pressure and an inspiratory time as opposed to a tidal volume to determine when to terminate a breath (Fig. 42-3). This allows the clinician to set a maximum pressure on a breath-by-breath basis that the ventilator will not exceed. This feature has become more popular with the increased awareness of ventilator-induced lung injury and the findings that high tidal volumes and airway pressure can exacerbate lung damage. Recent studies found that 25% of all patients receiving mechanical ventilation were in a PCV mode,47 thus defining the growing number of users.
The downside of PCV ventilation includes the inability to adjust to changing lung compliance, patient discomfort leading to increased sedation, and increased mean airway pressure. Rapid decreases in lung compliance can lead to substantial decreases in delivered minute volume.
In PCV ventilation, the parameters monitored are PEEP, inspiratory pressure, respiratory frequency, and the percent of the duty cycle (Ti/Ttot) spent in inspiration, commonly referred to as the i-time. In PCV, unlike VCV, no tidal volume or peak flow is set. Instead, tidal volume, minute volume, and peak flow are all determined by the compliance and resistance of the entire respiratory system. Modern ventilators do allow for the adjustment of the inspiratory slope (rise time) of each breath. This setting controls how fast the ventilator builds toward the preset pressure.5 In patients with a high inspiratory demand, a rapid rise time can improve synchronization. The inspiratory pressure refers to the delta-p, or change in pressure, independent of applied PEEP. This means that the total pressure is the sum of the inspiratory pressure and PEEP level, and that as PEEP is increased, delta-p remains the same and total pressure will increase. Some ventilators adjust this parameter automatically, while on others it is set by the operator.
PCV is the mode of choice when control of the maximum amount of alveolar pressure is the goal. This can limit barotrauma in at-risk patients. However, in the spontaneously breathing patient, transalveolar pressure can exceed alveolar pressures and drive ventilator-induced lung injury.43 This happens in spontaneously breathing patients in PCV mode. Decreasing the pleural pressure during a spontaneous breath may lead to an increase in the transalveolar pressure even though the maximum airway pressure is limited. Although one can limit maximum alveolar pressure in almost any ventilatory mode, with PCV, it is more intuitive and requires less user input.
Some clinicians prefer PCV because of the ability to easily control mean airway pressure (MAP). In PCV, the MAP can be affected by either adjusting the PEEP, the inspiratory pressure, or the inspiratory time. Frequency does not influence MAP (except in cases of pressure-control inverse ratio ventilation).99,100 MAP can be used as a guide to estimate alveolar pressures if inspiratory and expiratory resistance are equal. An exception to this rule is the patient with COPD. Such patients suffer from expiratory flow limitation (increased resistance to exhalation), leading to a MAP that underestimates the alveolar pressure.5
Even though numerous studies have touted the physiologic benefit of PCV over VCV, the clinical differences remain modest.59,86,87,88,89,90 Methodologic differences and flaws in study design have complicated their comparisons.91 While PCV continues to grow in popularity, so do variants of this type of ventilation.
Airway Pressure-Release Ventilation (APRV) and Inverse Ratio Ventilation (IRV)
APRV and IRV were developed as modes of ventilation to be used in extreme cases of hypoxia.92,93,94,95,96 The use of these modes requires a highly trained staff and neither of them has enjoyed widespread acceptance. Most studies have found little difference in mortality between these specialized modes and the more conventional methods of ventilation, although transient increases in PaO2 have been reported.42,149 Although several permutations of APRV now exist, including computer-controlled ventilator-driven algorithms, the basic pattern remains the same. High levels of continuous positive airway pressure (CPAP) are maintained with short periods of “dumping,” in which an exhalation valve rapidly release the CPAP. The CPAP level is quickly restored with fresh gas from the CPAP generator. The pattern of fill and dump can be selected by the operator and is based on mathematical modeling by Marini et al.98 Factors such as level of CPAP, speed of the dump cycle, time to recovery of CPAP level, as well as frequency of the cycle all influence effectiveness.
Variations of APRV exist under many names but all share common features. All involve essentially two levels of PEEP or CPAP that cycle at various frequencies. These modes—with names such as intermittent mandatory pressure-release ventilation, biphasic positive airway pressure, and bilevel PEEP—remain similar in principle. Key differences include the ability to allow spontaneous breathing or to apply pressure support to spontaneous breaths. Biphasic and bilevel ventilation usually allows for longer periods of time at the low CPAP level than does more traditional APRV.
In selecting one of the variations of APRV, the most important factor is the patient’s ability to breathe spontaneously as opposed to modes where spontaneous breathing is not allowed. Studies have shown a clear benefit to modes that allow for spontaneous breathing, including improved hemodynamics,71,126 improved oxygenation,126 improved ventilation/perfusion matching,58 and improved lung expansion and gas distrubution.101,102,103,104 While numerous studies have compared APRV to CMV,105,106,107,108 PCV,99,109,110,111 and high-frequency oscillatory ventilation (HFOV),67 no clear mortality benefit has been shown. Further studies demonstrating a mortality benefit are needed before there can be wide acceptance of this mode, which is complicated and requires careful attention to settings to prevent hemodynamic compromise.134
IRV was first described by Reynolds130 as a possible mode of ventilation in patients with acute lung injury. It was seen as a way to increase MAP while maintaining reasonable peak airway pressures. IRV can be used in either a pressure-controlled mode (PC-IRV) or a flow-controlled mode (VC-IRV), with the latter being far less popular.99,129 Early studies touted the improvement in gas exchange as evidence of efficacy of IRV in patients with ARDS.94,95,96,116,117 These early studies showing improved gas exchange frequently did not control for level of PEEP or MAP.
IRV frequently will result in intrinsic PEEP because of the stacking of breaths. Intrinsic PEEP results when inhalation begins prior to full exhalation of the previous breath. This “trapped gas” adds to the end-expiratory pressure, thus creating a higher level of PEEP than what is set on the ventilator (Figs. 42-4 and 42-5). This intrinsic PEEP can be measured by performing an expiratory hold, allowing for the gas in the lungs to equilibrate and then reading the resulting pressure from the ventilator. Some believe that intrinsic PEEP is a more selective form of PEEP favoring lung units with short time constants (as in ARDS), thus sparing healthy lung tissue with longer time
constants from cyclical opening and closing.81,168 Rather than being beneficial, intrinsic PEEP is counterproductive.59,120,121,122,123 Amato and Marini5 recommended that external PEEP should be applied to make IRV more effective. Because of the evidence suggesting that intrinsic PEEP can be harmful and the lack of outcome data showing a mortality difference with IRV, the use of this mode has declined in recent years. Many of the immediate benefits first observed with IRV were later shown not to be beneficial but rather harmful to the lung.
constants from cyclical opening and closing.81,168 Rather than being beneficial, intrinsic PEEP is counterproductive.59,120,121,122,123 Amato and Marini5 recommended that external PEEP should be applied to make IRV more effective. Because of the evidence suggesting that intrinsic PEEP can be harmful and the lack of outcome data showing a mortality difference with IRV, the use of this mode has declined in recent years. Many of the immediate benefits first observed with IRV were later shown not to be beneficial but rather harmful to the lung.
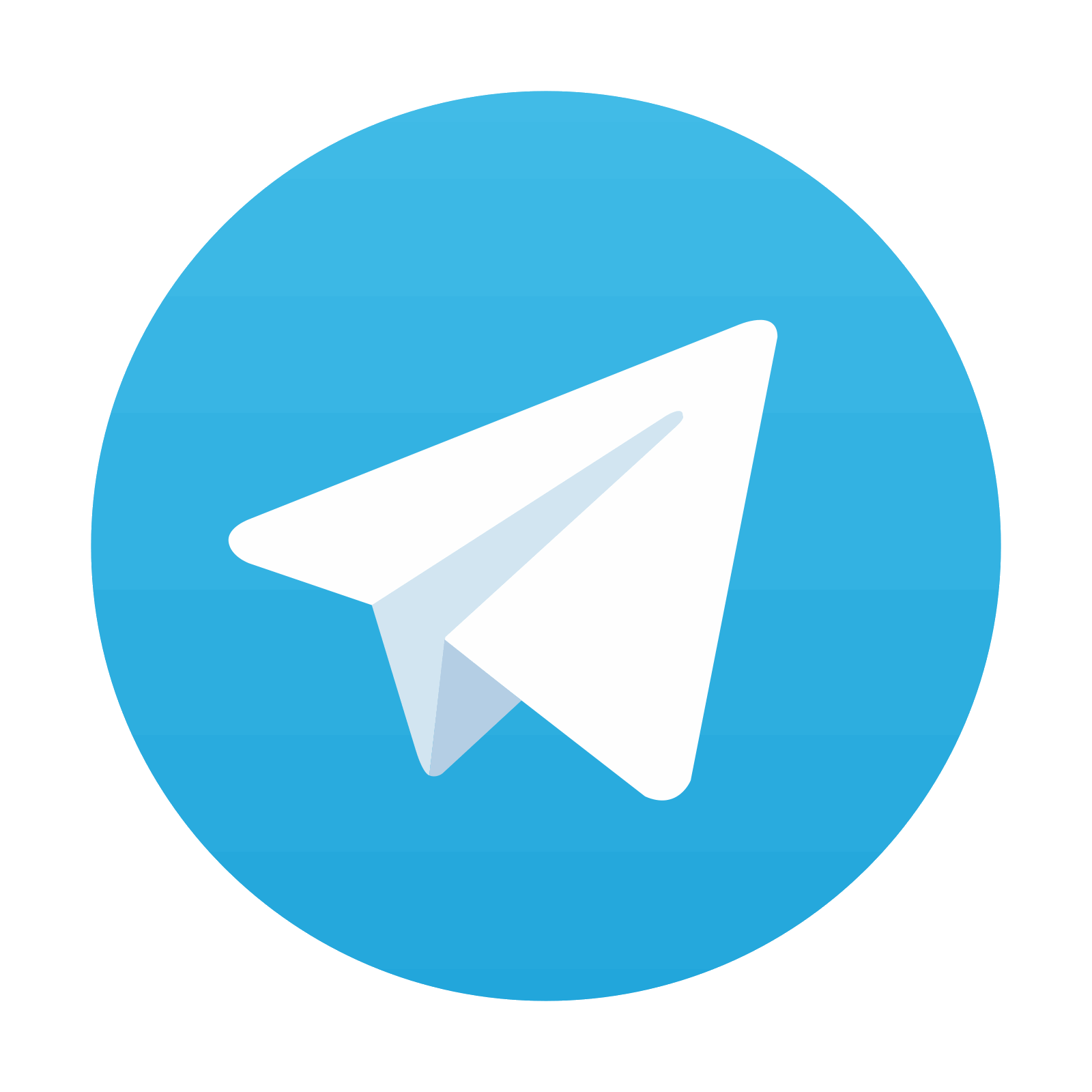
Stay updated, free articles. Join our Telegram channel
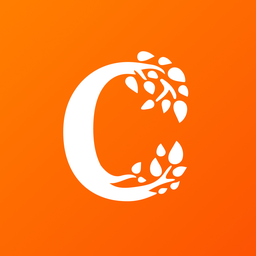
Full access? Get Clinical Tree
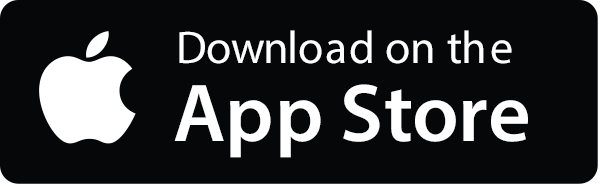
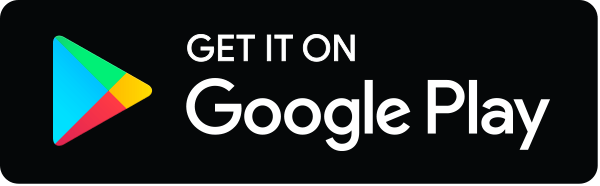