Introduction
Mechanical ventilation is the process of using a device (ventilator) to support, partially or totally the delivery of gas to the lungs. The desired effect of mechanical ventilation is to maintain adequate levels of P o 2 and P co 2 in arterial blood while also unloading the inspiratory muscles. Although negative-pressure chambers or wraps might fulfill this definition, this discussion focuses on the use of devices that use positive airway pressures.
Positive-pressure mechanical ventilation is used widely. In the United States, about 1 to 3 million patients annually are estimated to receive mechanical ventilatory support outside the operating room. Traditionally, this support has been provided in intensive care unit (ICU) settings, but there are clear trends toward expanding the venues to subacute facilities, long-term care facilities, and the home. As the number of elderly increases and as more aggressive surgical and immunosuppressive therapies are developed, the need for mechanical ventilation in all of these venues is likely to increase. In addition, increasing concerns about widespread outbreaks of respiratory pandemics has led many government agencies to stockpile large numbers of mechanical ventilators.
Positive-Pressure Mechanical Ventilator Design Features
Gas Delivery Systems
Positive-Pressure Breath Controller
Most modern ventilators utilize piston/bellows systems, turbines, or controllers of high-pressure sources to drive gas flow. Tidal breaths are generated by this gas flow and can either be controlled entirely by the ventilator or be interactive with patient efforts. Pneumatic, electronic, or microprocessor systems provide for various breath types. In general, these can be classified by what initiates the breath (trigger variable), what regulates gas delivery during the breath (target or limit variable), and what terminates the breath (cycle variable).
Breath triggers are generated by either a change in pressure or flow initiated by patient effort (assisted/supported breath) or by a set time (controlled breath). During the breath, gas delivery is regulated to meet a target or limit variable, which is generally either a set flow or a set inspiratory pressure. The breath is then terminated by a cycle variable, which can either be a set volume, a set inspiratory time, or a set flow. A high-pressure cycle variable is usually also present to limit lung over distention. Figure 101-1 uses this classification scheme to describe the five most common breath types available on the current generation of ventilators: volume assist (VA), volume control (VC), pressure assist (PA), pressure control (PC), and pressure support (PS).

Mode Controller/Feedback Systems
The availability and delivery logic of different breath types define the “mode” of mechanical ventilatory support. The mode controller is an electronic, pneumatic, or microprocessor-based system that is designed to provide the proper combination of breaths according to set algorithms and feedback data (conditional variables) ( Table 101-1 ).
BREATH TYPES AVAILABLE † | ||||||
---|---|---|---|---|---|---|
Mode | VC | VA | PC | PA | PS | Sp |
Volume assist—control | X | X | ||||
Pressure assist—control | X | X | ||||
Volume SIMV | X | X | X | X | ||
Pressure SIMV | X | X | X | X | ||
Pressure support | X |
* In addition to the five “basic” breaths depicted in Figure 101-1 , this table also includes spontaneous unassisted/unsupported breaths.
† VC, volume control; VA, volume assist; PC, pressure control; PA, pressure assist; PS, pressure support; Sp, spontaneous unassisted. SIMV, synchronized intermittent mandatory ventilation.
The simplest mode is assist-control ventilation (ACV), which can provide either flow-targeted volume-cycled breaths ( volume assist-control ventilation [VACV]) or pressure-targeted time-cycled breaths ( pressure assist-control ventilation [PACV]). A simple feedback system is employed with ACV that guarantees a set number of positive-pressure breaths. If the patient’s underlying respiratory rate exceeds this guarantee, all breaths are patient-triggered breaths (VA or PA breaths). If the patient’s respiratory rate is below this guarantee, the ventilator will “make up the difference” with mandatory (controlled) breaths (VC or PC breaths).
Another relatively simple mode is synchronized intermittent mandatory ventilation (SIMV), which can provide either flow-targeted volume-cycled breaths (volume SIMV) or pressure-targeted time-cycled breaths (pressure SIMV). Like ACV, SIMV guarantees a set minimal number of positive-pressure breaths. Unlike ACV, however, if the patient’s respiratory rate exceeds this guarantee, the ventilator will provide assisted breaths up to the set rate and then allow unassisted (simple SIMV mode) or flow-cycled pressure support breaths (SIMV + PS mode) thereafter. If the patient’s respiratory rate is below the set guarantee, the ventilator will again “make up the difference” with mandatory (controlled) breaths. Note that although PS breaths are often provided during SIMV, PS breaths can also be provided as a stand-alone mode without any guaranteed breaths (pressure support ventilation). Importantly, many modern systems also have algorithms to generate breaths when an apnea is detected; this represents a safety feature for modes with either low or no set guaranteed rates in case patient respiratory efforts are suddenly reduced or absent.
In recent years, more sophisticated feedback systems have been developed for these basic modes and are now available on many modern devices. These include pressure-regulated volume control (PRVC), volume support (VS), and adaptive support ventilation (ASV).
PRVC (also known by proprietary names such as “VC+,” “Autoflow,” and others) is an assist-control PACV that uses tidal volume as a feedback control for continuously adjusting the pressure target. The clinician sets a tidal volume target, and the ventilator then automatically sets the inspiratory pressure within a clinician-set range to achieve this goal.
VS is also based on a feedback design using patient-triggered, pressure-targeted, flow-cycled PS breaths. If a patient’s respiratory drive exceeds the clinician-set guaranteed rate, some PRVC systems will provide additional patient-triggered, time-cycled PRVC breaths while others will provide patient-triggered flow-cycled VS breaths. It is important to note that, with both PRVC and VS breaths, an improvement in respiratory mechanics will result in a lower applied inspiratory pressure, whereas a worsening in respiratory mechanics will result in a higher applied inspiratory pressure. Similarly, increasing respiratory effort by the patient will result in a lower applied inspiratory pressure, whereas decreasing respiratory effort by the patient will result in a higher applied inspiratory pressure.
ASV is also an assist-control, pressure-targeted, time-cycled mode of ventilation PACV that utilizes respiratory system mechanics to set the tidal volume-frequency pattern. The clinician sets only a desired minute ventilation and patient weight (for estimating anatomic dead space). Using controlled breaths, ASV initially calculates resistance and compliance, as well as the expiratory time constant (resistance × compliance). The ASV algorithm then adjusts the frequency–tidal volume pattern to minimize ventilator work (integral of pressure over volume) and thus conceptually to minimize applied forces to the lungs. The breathing pattern is also modulated by incorporating the expiratory time constant to avoid air trapping. As respiratory mechanics change, the frequency–tidal volume pattern is automatically adjusted to maintain minimal ventilatory work. In contrast, if patient efforts are triggering breaths with ASV, it behaves much like VS.
Subsystems of Mechanical Ventilators
Effort (Demand) Sensors
Current ventilators have sensors that detect patient effort, thus allowing for a number of interactions between the patient and the ventilator. Examples include patient-triggered breaths in which the ventilator initiates flow in response to patient demand and pressure-targeted/limited breaths in which the ventilator adjusts flow in response to patient demand (see “ Patient-Ventilator Interactions ” later). These sensors are usually either pressure or flow transducers in the ventilatory circuitry and are characterized by their sensitivity (the circuit pressure or flow change needed to initiate a ventilator response) and their responsiveness (the time needed to provide this response).
Gas Blenders
Blenders mix air and O 2 to produce a fractional concentration of O 2 in inspired gas (F io 2 ) ranging from 0.21 to 1.0. On newer systems, blenders are also available for other gases such as heliox, nitric oxide (NO), and anesthetic agents.
Humidifiers
With the upper airway bypassed by tracheal intubation, sufficient heat and moisture must be added to the inspired gas mixtures to avoid mucosal desiccation. Active humidifiers utilize external water sources and electrical power to adjust blended gas mixtures to near body conditions (tracheal temperature of > 35° C, water content of > 40 mg/L). Heated wire circuits help facilitate this by preventing condensation and “rainout” in the ventilator tubing. Passive humidifiers use simple heat/moisture exchange devices in the ventilator circuit that reutilize heat and moisture trapped from expired gas. These disposable units can usually supply adequate heat and moisture (i.e., > 30° to 33° C and > 28 to 32 mg/L H 2 O) for many patients, particularly those receiving mechanical ventilation for only short periods of time.
Expiratory Pressure Generator
Positive end-expiratory pressure (PEEP), or positive airway pressure throughout expiration, can be generated to help maintain alveolar patency and improve ventilation-perfusion ( ) matching (see “ Physiologic Effects of Positive-Pressure Mechanical Ventilation ” later). PEEP is usually applied by regulating pressure in the expiratory valve of the ventilator system but can also be applied by providing a continuous flow of source gas during the expiratory phase. Some expiratory valves have measurable resistance even when fully open; this may result in inadvertent PEEP. As discussed in more detail later, a positive alveolar pressure may be present at end expiration if the expiratory time is inadequate for the lung to return to its “resting volume” or if significant flow limitation exists. Such inadvertent PEEP is referred to as intrinsic PEEP (PEEPi), or as “auto-PEEP,” “occult PEEP,” or “air trapping.”
Gas Delivery Circuit
The circuit linking the ventilator to the patient usually consists of flexible tubing that often contains pressure or flow sensors, closed suctioning systems, and an exhalation valve. It is important to remember that, because this tubing has measurable compliance (2 to 4 mL/cm H 2 O are representative values), high circuit pressures may induce significant amounts of delivered gas to distend the circuitry rather than to enter the patient’s lungs.
Patient–Ventilator Circuit Interface
Positive-pressure ventilation is usually delivered through a tube inserted into the patient’s airway (orotracheal or nasotracheal tube or tracheostomy). These tubes generally have air-filled balloons, which are inflated to provide a proper airway seal. An alternative to the tracheal tube is a mask system. Both full-face and nasal masks have been utilized with a variety of ventilatory support systems and modes. Leaks around masks, however, can be significant and thus ventilatory support modes using masks must be able to provide adequate volumes and proper inspiratory timing. To this end, special mask ventilators with pressure-targeted and either time-cycled or leak-compensated, flow-cycled capabilities have been developed.
Aerosol Generators (see Chapter 11 )
Therapeutic aerosols (e.g., bronchodilators, steroids, vasodilators, antibiotics) can be delivered through the ventilator circuitry either by in-line nebulizers or by special adapters designed for metered-dose inhalers. Lung deposition is generally less in an intubated than in a nonintubated patient because the endotracheal tube serves as a significant barrier to aerosol delivery. Higher dosing is thus advisable.
The location of the aerosol generator in the ventilator circuit can affect deposition. The optimal site appears to be in the inspiratory limb several centimeters proximal to the patient’s “wye” connector. This location allows either nebulized medications or a metered-dose actuation to “charge” the inspiratory limb of the circuit during exhalation. Aerosol particle velocity is also slowed and becomes the leading portion of the next inspiration, both of which facilitate delivery.
Monitors and Graphic Displays
Although electronic and microprocessor-based systems have considerable internal monitoring of electronic and pneumatic function, the three variables generally displayed for clinical use are circuit pressures, flows, and volumes. Pressure sensors in the esophagus to estimate pleural pressures are also available. Alarms can be utilized on all of these monitors. Importantly, trending capabilities for up to 72 hours or more are now available on many modern systems. Most modern positive-pressure ventilators also have oxygen sensors in the circuitry to ensure that the desired F io 2 is being delivered. In addition, some ventilators may also have analyzers for measuring exhaled carbon dioxide and inhaled therapeutic gases such as NO or heliox.
Physiologic Effects of Positive-Pressure Mechanical Ventilation
Ventilation and Respiratory System Mechanics (see Chapter 5 )
Alveolar Ventilation and the Equation of Motion
Alveolar ventilation is the term for the delivery of fresh gas to the gas exchange regions of the lungs. Mathematically, this is expressed as:





Driving pressure = ( flow × resistance ) + ( volume/system compliance )


During an inspiratory hold at end-inspiration in a patient who is not making respiratory efforts (i.e., no-flow condition: = 0, Pmus = 0), the ventilator circuit pressure “plateaus” at a pressure commonly referred to as the plateau pressure (Pplat). In this way, the components of Pcir can be determined. Specifically, the difference in Pcir during flow and during no-flow (the “peak to plateau difference”) allows for a calculation of total inspiratory resistance:
R = ( Ppeak − Pplat ) / V ˙




Flow-Targeted Versus Pressure-Targeted Breaths
There are two basic approaches to delivering positive-pressure breaths: flow targeting and pressure targeting (see Fig. 101-1 ). With flow targeting (breaths 1 and 2 in Fig. 101-1 ), the clinician sets the inspiratory flow; circuit pressure is then the dependent variable. With pressure targeting (breaths 3 through 5 in Fig. 101-1 ), the clinician sets an inspiratory pressure target (with either time or flow as the cycling criterion); flow and volume are then the dependent variables (i.e., varying with the lung mechanics and patient effort). With a flow-targeted breath, changes in compliance, resistance, or patient effort will change Pcir (but not flow); in contrast, with a pressure-targeted breath, similar changes in compliance, resistance, or effort will cause a change of tidal volume (but not Pcir) (see dashed lines in Fig. 101-1 ).
Each strategy has advantages. For flow-targeted breaths, a minimal tidal volume is guaranteed. For pressure-targeted breaths, the rapid initial flow and subsequent adjustable flow of pressure targeting may enhance gas mixing and patient synchrony (see discussions “ Distribution of Ventilation ” and “ Patient-Ventilator Interactions ” later).
Pressure-targeted breaths can also be modified with a volume feedback feature described earlier for PRVC and VS breaths to combine the enhanced gas mixing and patient-ventilator synchrony effects of a pressure-targeted breath with a certain volume guarantee. However, it is important to realize that providing a volume guarantee negates the pressure-limit feature set by the clinician because, with PRVC/VS, worsening respiratory system mechanics will drive applied pressures up. Another potential problem with PRVC/VS is that it may not adequately unload the patient if patient effort inappropriately increases due to pain or anxiety.
PEEPi and the Ventilatory Pattern
PEEPi is the positive alveolar end-expiratory pressure that arises because of inadequate expiration, caused by either inadequate expiratory time or airway collapse during expiration (or both). PEEPi increases with increased minute ventilation, decreased expiratory time fraction, and the increased respiratory system expiratory time constant (the product of resistance and compliance).
The development of PEEPi will have different effects on flow-targeted compared with pressure-targeted ventilation. In flow-targeted ventilation, the constant delivered flow and volume (and thus ΔPcir) in the setting of a rising PEEPi will increase both the Ppeak and the Pplat. In contrast, in pressure-targeted ventilation, the set Pcir limit coupled with a rising PEEPi level will decrease ΔPcir and thus the delivered tidal volume (and minute ventilation). Importantly, this may help limit the build-up of PEEPi.
In the patient without respiratory effort, PEEPi can be recognized in two ways. First, when PEEPi is produced by an inadequate expiratory time, analysis of the flow graphic will show that expiratory flow has not returned to zero before the next breath is given. Second, PEEPi in alveoli with patent airways can be quantified during an expiratory hold maneuver that permits equilibration of the PEEPi with Pcir.
In the patient with active respiratory effort, PEEPi can be assumed to be present if the expiratory flow has not reached zero before the next breath begins. However, the expiratory hold maneuver cannot be interpreted in an actively breathing patient. As noted in more detail later, in an actively breathing patient, PEEPi can function as an inspiratory threshold load. This is best quantified by using Pes to estimate pleural pressures. With this technique, the change in Pes before a change in Pcir is a reflection of the threshold load imposed by PEEPi (see “ Patient-Ventilator Interactions ” later).
Distribution of Ventilation
A positive-pressure tidal breath must be distributed among the millions of alveolar units in the lung. Factors affecting this distribution include patient-related factors: regional resistances, compliances, and functional residual capacities. Breaths will tend to distribute more to units with high compliance and low resistance and away from obstructed or stiff units ( Fig. 101-2A , Low Regional C l ). This creates the potential for regional overdistention of healthier lung units, even in the face of “normal”-sized tidal volumes (see “ Ventilator-Induced Lung Injury ” later).

The flow pattern set on the ventilator may also affect ventilation distribution. For example, when there are marked inhomogeneities in airway resistance, slow and constant flows will tend to distribute gas more evenly (although consequent shorter expiratory times may worsen air trapping). In addition, inspiratory pauses can also allow pendelluft action to fill slowly filling alveoli ( Fig. 101-2B , High Regional R aw ). In contrast, when there is parenchymal lung injury with minimal inhomogeneities in airway resistance, initially rapid flows with subsequent deceleration (typically seen in pressure-targeted breaths) may distribute gas more evenly and will pressurize lung units rapidly, producing a higher mean inspiratory alveolar pressure for a given breath volume.
It should be noted that more uniform ventilation distribution does not necessarily mean better matching (i.e., more homogeneous ventilation distribution may actually worsen
matching in a lung with inhomogeneous perfusion). Because of all these considerations, predicting which flow pattern will optimize ventilation-perfusion matching is difficult and often requires trial and error.
Alveolar Recruitment and Gas Exchange
Because of alveolar flooding, inflammatory exudates, and collapse, parenchymal lung injury leads to mismatching and shunts. In many (but not all) of these disease processes, substantial numbers of collapsed alveoli can be recruited during a positive-pressure ventilatory cycle. Additional recruitment can sometimes be provided with the use of formal recruitment maneuvers or prolongation of inspiratory time. The application of PEEP is designed to prevent derecruitment during exhalation.
Recruitment Maneuvers
Recruitment maneuvers (RMs) can be performed using sustained inflations (e.g., 30 to 40 cm H 2 O) for up to 30 to 120 seconds, by transient elevations of the PEEP-tidal volume settings, and by single or multiple “sighs” that take the lung briefly to near total lung capacity. To avoid patient inspiratory or expiratory efforts, additional sedation or even neuromuscular blockade may be used. Importantly, RMs can have adverse hemodynamic consequences; close monitoring of the patient is mandatory during RMs. RMs provide initial alveolar recruitment only—maintenance of recruitment requires setting PEEP appropriately to prevent subsequent derecruitment.
Inspiratory Time Prolongations
A positive-pressure breath produces a flow magnitude and a flow profile that, as noted previously, can affect ventilation distribution (and thus ). Prolonging inspiratory time, generally by adding a pause, often in conjunction with a rapid decelerating flow (i.e., pressure-targeted breath), has several physiologic effects. First, the longer inflation period may recruit more alveoli. Second, increased gas mixing time may improve
matching in parenchymal lung injury (pendelufft). Third, the development of PEEPi from consequently shorter expiratory times can have effects similar to those of applied PEEP (see earlier). It should be noted, however, that the distribution of PEEPi, which is most pronounced in lung units with long expiratory time constants, may be different from that of applied PEEP and thus the effects on
may also be different with intrinsic compared with applied PEEP. Fourth, because these long inspiratory times significantly increase total intrathoracic pressures, cardiac output may be reduced (see “ Positive-Pressure Ventilation and Cardiac Function ” later). And finally, inspiratory-to-expiratory ratios that exceed 1 : 1 (so-called inverse-ratio ventilation) are uncomfortable, and patient sedation/paralysis is often required unless a relief mechanism allows spontaneous breathing during the inflation period (see “ Airway Pressure Release Ventilation ” later).
Positive End-Expiratory Pressure
PEEP is defined as an elevated airway pressure at the end of expiration. As noted earlier, PEEP can be produced by either expiratory circuit valves (applied PEEP) or inadequate expiratory times in lung units with long expiratory time constants (PEEPi). Note that expiratory muscle contraction can also raise intrathoracic pressures at end-expiration, but this does not have the same effects on the lungs because transpulmonary pressure is not increased.
PEEP helps to recruit or maintain alveolar units open, providing several potential benefits. First, recruited alveoli improve matching and gas exchange throughout the ventilatory cycle. Second, as discussed in more detail later, patent alveoli throughout the ventilatory cycle are not exposed to the risk of injury from the shear stress of repeated opening and closing. Third, open alveoli with intact surfactant monolayers improve lung compliance. This is the rationale behind applying PEEP after an RM: recruited alveoli are on the deflation limb of the pressure-volume relationship and thus the pressure required to maintain recruitment is lower than that required for initial recruitment.
PEEP, however, can also be detrimental. Because the tidal breath is delivered on top of the baseline PEEP, end-inspiratory pressures are usually raised by the application of PEEP (although this increase may be less than the actual added PEEP because of improved compliance). This increase must be considered if the lung is at risk for regional overdistention (see “ Ventilator-Induced Lung Injury ” later). Moreover, since parenchymal lung injury is often quite heterogeneous, appropriate PEEP in one region may be suboptimal in another and excessive in yet another. Optimizing PEEP is thus a balance between recruiting the recruitable alveoli in diseased regions without overdistending already recruited alveoli in healthier regions. Another potential detrimental effect of PEEP is that it raises mean intrathoracic pressure, thereby compromising cardiac filling in susceptible patients (see “ Positive-Pressure Ventilation and Cardiac Function ” section later).
Mechanical Loads
Mechanical loads describe the physical requirements of ventilation with a single value, either the pressure-time product (PTP—the integral of pressure over time) or work (W—the integral of pressure over volume). Because mechanical loads correlate with inspiratory muscle oxygen demands, the concept of load is useful in considering inspiratory muscle energy requirements during spontaneous or interactive ventilatory support. Moreover, as described in more detail later, load referenced to muscle strength and/or endurance properties (e.g., PTP or W divided by muscle pressure-generating capability) can be used to set levels of partial ventilatory support or predict spontaneous breathing capabilities.
Compliance, resistance, flow, and volume all contribute to the magnitude of the load per breath. During spontaneous breaths, Pcir is zero and integrating Pes over time or volume (referenced to the passive inflation pressure) describes the load borne by the inspiratory muscles to inflate the lungs. During a controlled breath, integrating Pcir over time or volume describes the load borne by the ventilator to inflate the entire respiratory system (lungs and chest wall) and integrating Pes over time or volume describes the loads imposed by the chest wall only. During interactive breaths the load is shared between patient and ventilator.
Under heavy loading conditions (e.g., the patient with abnormal respiratory system mechanics and thus high pressure requirements), the duration of pressure (i.e., the PTP) correlates better with muscle energetics and fatigue potential than does the volume moved with pressure (i.e., work). Indeed, during ventilation requiring high pressures, multiplying PTP by the inspiratory time fraction and referencing this to the maximal pressure that the inspiratory muscles can generate results in the pressure-time index (PTI). Muscle fatigue can be expected if the PTI value exceeds 0.15. The concern with high pressure loads in patients receiving partial ventilatory support is one of the rationales for providing ventilator pressure assistance with every spontaneous effort (i.e., pressure-assisted or supported breaths), as opposed to supporting only some breaths as in intermittent mandatory ventilation without pressure support (see “ Patient-Ventilator Interactions ” later).
Inspiratory muscle overload is one of the major determinants of continuing ventilator dependency and can result either from excessive mechanical loads or from inspiratory muscle dysfunction. Excessive mechanical loads can result from disease or from inappropriate ventilatory assistance (see “ Patient-Ventilator Interactions ” later). Clinically, inspiratory muscle overload is manifested by rapid, shallow breathing patterns, paradoxical abdominal motion, and patient distress. Inspiratory muscle dysfunction can be a result of the systemic inflammatory response syndrome, metabolic disturbances, drugs (e.g., steroids, previous use of neuromuscular blockers), malnutrition, or malpositioning (i.e., diaphragm flattening from lung overinflation). Finally, insufficient loading may also affect inspiratory muscles. Specifically, controlled mechanical ventilation without any patient effort, perhaps for as little as 24 hours, may produce muscle changes similar to disuse atrophy—a condition described as ventilator-induced diaphragmatic dysfunction (VIDD).
Patient-Ventilator Interactions
Mechanical ventilation modes that permit spontaneous ventilatory activity are termed “interactive” modes. These interactions can range from simple triggering of mechanical breaths to more complex processes affecting delivered flow patterns and breath timing. Interactive modes allow for muscle “exercise,” which, when performed at nonfatiguing or physiologic levels, may prevent VIDD and facilitate fatigue recovery. In addition, permitting spontaneous patient ventilatory activity with “comfortable” interactive modes may reduce the need for the sedation and/or neuromuscular blockers that may otherwise be used to prevent patients from “fighting the ventilator.” Interactions take place during all three phases of breath delivery: breath triggering, flow delivery, and breath cycling and are described in detail later in “Patient-Ventilator Dys-synchrony.”
Positive-Pressure Ventilation and Cardiac Function
In addition to affecting ventilation and ventilation distribution, positive-pressure ventilation can also affect cardiovascular function. In general, as mean intrathoracic pressure increases, right ventricular filling decreases and cardiac output/pulmonary perfusion consequently decreases. This is the rationale for using volume repletion to maintain cardiac output in the setting of high intrathoracic pressure. Of note, the effect of reduced cardiac filling on cardiac output may be partially counteracted by improvement in left ventricular function due to elevated intrathoracic pressure, which can reduce left ventricular afterload. Importantly, in patients with left heart failure, the reduced cardiac filling and reduced left ventricular afterload effects of elevated intrathoracic pressure may improve cardiac function to the point that the removal of intrathoracic pressure may worsen cardiac function and thereby produce weaning failure.
Intrathoracic pressures can also influence distribution of perfusion. The relationship of alveolar pressures to perfusion pressures in the three-zone West lung model helps explain this. Specifically, the dependent lung is generally in a zone 3 (capillary distention) state. As intra-alveolar pressures rise, however, zone 2 and zone 1 (capillary collapse/dead space) regions can appear, creating high units. Indeed, increases in dead space can be a consequence of ventilatory strategies employing high ventilatory pressures, as well as with those producing PEEPi.
Positive-pressure mechanical ventilation can affect other aspects of cardiovascular function. Specifically, dyspnea, anxiety, and discomfort from inadequate ventilatory support can lead to stress-related catecholamine release with subsequent increases in myocardial oxygen demands and risk of dysrhythmias. In addition, coronary blood vessel oxygen delivery can be compromised by inadequate gas exchange from the lung injury coupled with low mixed venous P o 2 due to high oxygen consumption demands by the inspiratory muscles.
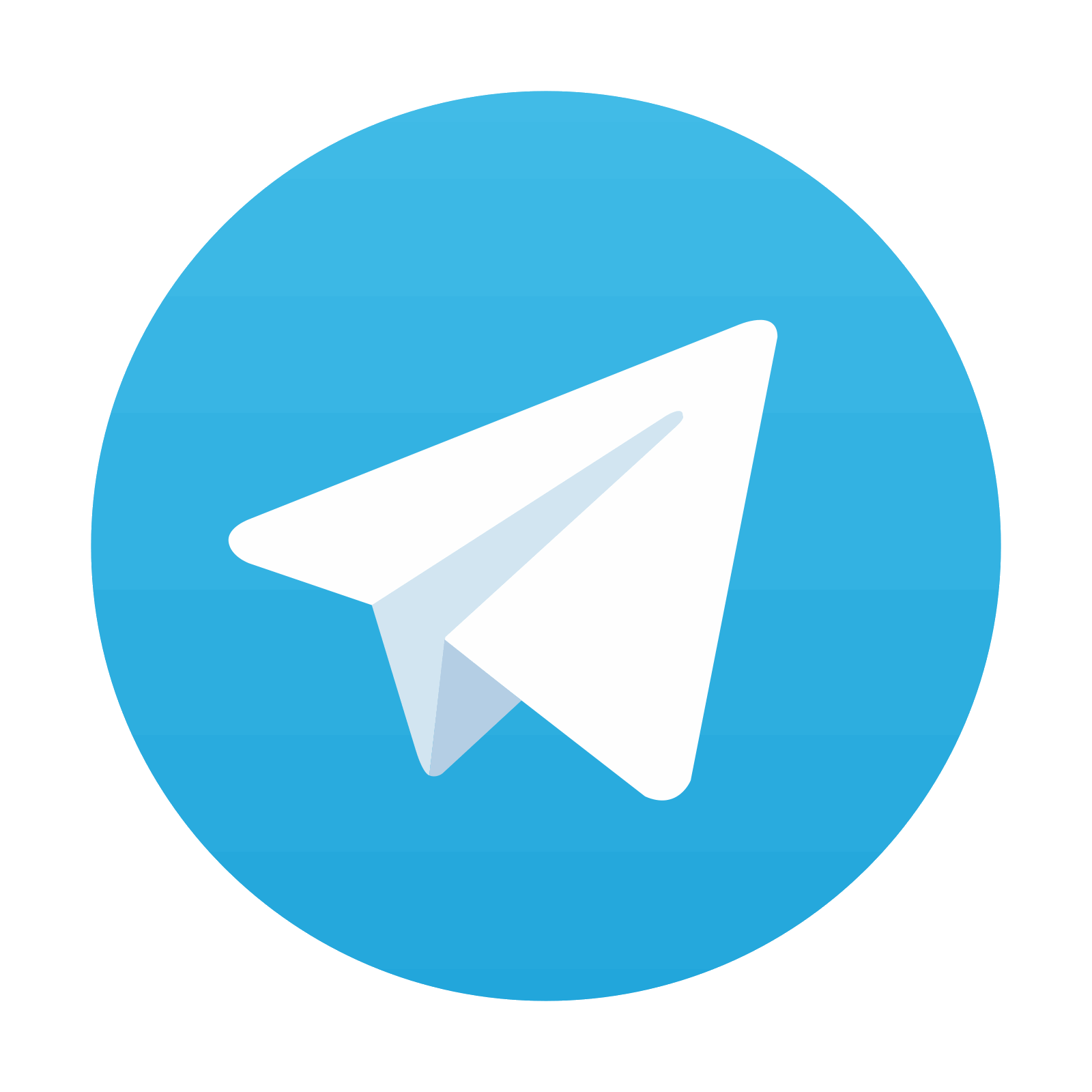
Stay updated, free articles. Join our Telegram channel
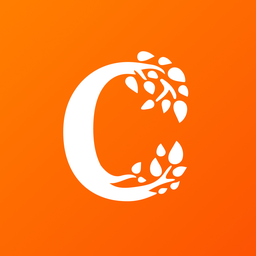
Full access? Get Clinical Tree
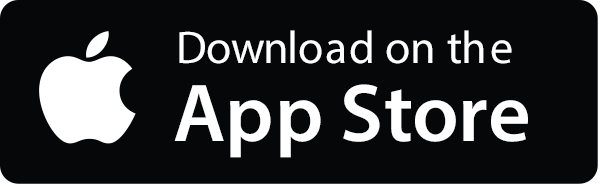
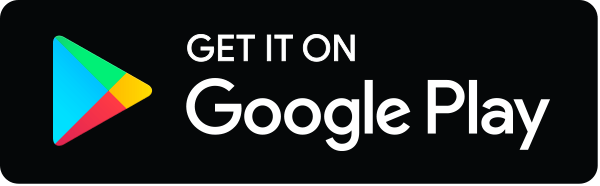