Chapter 13
Lymphatic Pathophysiology
Marlys H. Witte, Michael J. Bernas
Dedicated to the memory of Charles L. Witte, MD (1935-2003), beloved husband and father, and great teacher, researcher, and clinician. He was the primary author of the previous version of this chapter and an internationally recognized authority in the field of lymphology.
In a narrow sense, the lymph circulation is a unidirectional vascular system that merely transports surplus tissue fluid back to the bloodstream. In a broader sense, however, this network stabilizes the mobile intercellular liquid and extracellular matrix microenvironment to ensure parenchymal cellular integrity and function.
In its entirety, the lymphatic system is composed of vascular conduits: lymphoid organs, including the lymph nodes, spleen, Peyer’s patches, thymus, and nasopharyngeal tonsils; and cellular elements that circulate in the liquid lymph, such as lymphocytes and macrophages. These migrating cells cross the blood-capillary barrier along with a multitude of immunoglobulins, polypeptides, plasma protein complexes, and cytokines and enter the lymphatics to return to the bloodstream. Although body water circulates very rapidly as a plasma suspension of red blood cells within the blood vascular compartment, it percolates slowly outside the bloodstream as a tissue fluid-lymph suspension of lymphocytes through lymph vessels and lymph nodes. As a specialized subcompartment of the extracellular space, therefore, the lymphatic system completes a closed loop for the circulation by returning liquid, macromolecules, and other blood elements that “escape” or “leak” from blood capillaries (Fig. 13-1).
Figure 13-1 Blood-lymph circulatory loop. Within the bloodstream, liquid flows rapidly as a plasma suspension of erythrocytes; outside the bloodstream, it flows slowly as a tissue fluid-lymph suspension of immunocytes through the lymphatics and lymph nodes. Small and large molecules, including plasma protein, trafficking cells, particulates, and respiratory gases, cross the blood-capillary endothelial barrier, percolate through the tissues, enter the lymph stream, and return to the central venous system to complete the loop. Clinical disorders of the blood-lymph circulatory loop are manifested as swelling, scarring, immunodeficiency, nutritional depletion, and uncontrolled lymphangiogenesis and angiotumorigenesis. Current and potential future therapeutic approaches are in parentheses.
Disruption of this blood-lymph loop promotes tissue swelling and is also responsible for a variety of syndromes characterized by scarring, wasting, immunodeficiency, and disordered angiogenesis. Rational therapeutic approaches, now and in the future, should be based on current and expanded understanding of these fundamental pathophysiologic principles.
Anatomy
Although, historically, identification of lymphatic vessels has long been hampered by the lack of readily identifiable structures, early physicians from Hippocrates (460-377 BC) to Aristotle (384-322 BC) and Erasistratus (310-250 BC) did describe vessels and nodes and on occasion noted visible intestinal lymphatic vessels in recently fed animals (see Kanter1 for more details). After a period of little scientific advancement, the discovery of chylous mesenteric lacteals in a well-fed dog by Gasparo Asellius early in the 17th century set off a flurry of anatomic dissections in England and continental Europe that established the nearly ubiquitous presence of lymphatics throughout the body and their important role in the absorption of nutrients (Box 13-1).2 These lymphatic “absorbents” accompany venous trunks everywhere, except in the central nervous system and the cortical bony skeleton.
Macroscopic Anatomy
In general, lymph from the lower part of the torso and viscera enters the bloodstream via the thoracic duct at the left subclavian-jugular venous junction (see Figs. 13-1 and 13-2). Lymphatics from the head and neck and from the upper extremities enter the central veins either independently or by a common supraclavicular cistern. Numerous interconnections exist within this rich vascular network, and subvariant anatomic pathways are plentiful. For example, the bulk of cardiac and pulmonary lymph, as well as intraperitoneal fluid, which drains through fenestrae of the diaphragm into substernal mediastinal collectors, unite as a common trunk to empty into the great veins in the right side of the neck. In contrast, intestinal lymph, which transports cholesterol, fat-soluble vitamins (vitamins A, D, K, and E), and long-chain triglycerides as chylomicra, courses retroperitoneally to the aortic hiatus, to form with other visceral and retroperitoneal lymphatics, the multichannel cisterna chyli and the thoracic duct, unlike intestinal blood, which flows directly into the liver. The bulk of the lymph formed in the liver flows retrograde or countercurrent to portal blood flow and joins intestinal lymph collectors just before the origin of the thoracic duct. Only a small amount of hepatic lymph drains anterograde along the major hepatic veins to the anterior mediastinum and right lymph duct. Although these topographic variants influence the development and progression of peripheral (lymph)edema only indirectly, they are nonetheless essential for a broad understanding of edema syndromes, including those accompanied by visceral lymphatic abnormalities, celomic effusions, and chylous reflux.
Figure 13-2 Macroscopic anatomy of the lymphatic system including major vessels, structures and nodes in relation to major arteries and veins. 1: left internal jugular vein; 2: left subclavian vein; 3: thoracic duct; 4: parotid lymph nodes; 5: submandibular lymph nodes; 6: accessory and comitant lymph nodes of the accessory nerve; 7: internal jugular lymph nodes with left jugular trunk; 8: supraclavicular lymph nodes with left supraclavicular trunk; 9: axillary lymph nodes with left subclavian trunk; 10: intercostal lymph nodes with left intercostals trunk; 11: parasternal lymph nodes with parasternal trunk; 12: anterior mediastinal lymph nodes with left anterior mediastinal trunk; 13: tracheobronchial lymph nodes with left tracheobronchial trunk; 14: cisterna chyli; 15a: left lumbar trunk; 15b: right lumbar trunk; 16: mesenteric lymph nodes; 17: lumbar lymph nodes; 18: left common iliac lymph nodes; 19: right external iliac lymph nodes; 20: internal iliac; 21: inguinal lymph nodes; 22: right lymphatic duct. (Modified from Kubik S: In Földi M, Földi E, editors: Foldi’s textbook of lymphology, ed 3, Munich, 2012, Urban & Fischer.)
Although the retina and brain do not technically have lymphatic apparatuses, they possess analogous circulations, such as the aqueous humor canal of Schlemm (the anterior chamber of the eye) or the cerebrospinal fluid/subarachnoid villus (pacchionian bodies) connections (the brain). Glial elements and non–endothelial-lined intracerebral perivascular (Virchow-Robin) spaces probably also serve to transport interstitial fluid to nearby intracranial venous sinuses. Extensive interruption of the cervical lymphatic trunks (e.g., after bilateral radical neck dissection) therefore causes prominent facial suffusion and a transient neurologic syndrome resembling pseudotumor cerebri,3 whereas an infusion of crystalloid solution directly into the canine cisterna magna causes an elevation in intracranial pressure and increases lymph flow from draining neck lymphatics.4,5 Although abundant lymphatic pathways thus exist for surplus tissue fluid to return to the bloodstream, homeostasis of the internal environment nonetheless still depends on an unimpeded, intact, interstitial-lymph fluid circulatory system (see Figs. 13-1 and 13-2).
Embryonic Development
Controversy has persisted since the early 1900s about the embryologic origin of lymphatics, that is, lymphovasculogenesis (endothelial precursors or stem cells, such as lymphangioblasts, differentiate and proliferate into a primitive tubular network) and subsequent lymphangiogenesis (sprouting from existing vessels) (Box 13-1).6 According to Sabin, lymphatics and veins derive from a common primordium in the venous system (Fig. 13-3).7 After injection of dye into pig skin, gradually extending lymphatic plexuses were observed, starting first at the base of the neck, which were in direct communication with the venous system. Sabin concluded that the primary lymphatic plexuses derive from central veins and that their growth progresses centrifugally by sprouting toward the periphery and ultimately forming the superficial lymphatic system. In contrast, Kampmeier,8 after a review of serial tissue sections, including Sabin’s original human embryo preparations, and phylogenetic considerations, proposed the centripetal theory that the lymphatic system arises independently from tissue mesenchyme in peripheral tissues and the surrounding primary lymph sacs and only later joins the central venous system to complete the blood-lymph loop. Kampmeier reasoned further that as the developing blood capillaries begin to “leak clear fluid into tissues, lymph amasses in ever growing volume within the mesenchyme first collecting in separate pools or spaces, which then become confluent as the high pressure stream seeks an outlet…the current usurps the course of decadent and vanishing venules, expands and pours into the developing jugular lymph sac, which meanwhile has acquired continuity with the forming arch of the cardinal lymphatic. Thoracic and abdominal lymphatics form similarly: Discontinuous spaces spring up in the mesenchyme next to the intercardinal or periaortic venous plexus in the path of the potential lymphatic; and primordia quickly widen, lengthen and fuse into a continuous vessel.”8
Figure 13-3 The developing lymphatic system showing the primary lymph sacs and the thoracic duct primordium, as well as several lymph nodes of a 2-month-old human embryo. (Modified from Sabin FR: On the origin and development of the lymphatic system from the veins and the development of the lymph hearts and the thoracic duct in the pig. Am J Anat 1:367, 1902; and Yoffey JM, et al: Lymphatics, lymph and the lymphomyeloid complex, New York, 1970, Academic Press.)
Although recent studies of the growth regulatory gene PROX19 and the receptor for lymphatic vascular endothelial growth factor (VEGFR-3)10 have tended to support the centrifugal theory (origin from venous spouting), other elegant work has demonstrated a substantial centripetal contribution from mesenchymal lymphangioblasts in the engrafted wing lymphatic system of chimeric quail chick embryos11 and early avian embryos through PROX1 staining.12 Both processes may contribute in various degrees to the ultimate link between the lymph and blood vasculature (e.g., the cervical thoracic duct/venous junction in humans). In addition, similar processes of lymphovasculogenesis and lymphangiogenesis are recapitulated after birth and probably involve the same complex expanding cascade of vascular growth factors (particularly the VEGF and angiopoietin families), endothelial receptors, and transcription factors already implicated in lymphatic growth and lymphedema-angiodysplasia syndromes (Table 13-1) (see the section on Lymphangiogenesis).
Light Microscopic Anatomy
As an afferent vascular system, the lymphatics originate within the interstitium as specialized capillaries, although in certain organs, such as the liver, they seem to emanate from nonendothelialized precapillary channels (e.g., the spaces of Disse).13 Lymphatic capillaries are remarkably porous and readily permit the entry of even large macromolecules (molecular weight >1000 kD). In this respect, they resemble the uniquely “leaky” fenestrated sinusoidal blood capillaries of the liver, but are in distinct contrast to most other blood capillaries, which are relatively impervious to macromolecules even the size of albumin (molecular weight, 69 kD).14
Under light microscopy without pre–paraffin-embedded tracer or intravascular latex injection, it is difficult to distinguish between blood and lymph vessels, although the latter are usually thin walled and tortuous, have a wider, more irregular lumen, and are largely devoid of red blood cells. Many staining features have been advocated to differentiate between blood and lymph microvasculature, such as the endothelial marker factor VIII–related antigen : von Willebrand factor (vWF). Although staining characteristics vary in both normal and pathologic states and at different sites (perhaps related to endothelial cell de-differentiation), in general, lymphatic staining resembles but is less intense than its blood vessel counterpart. In other words, the staining differences have been more quantitative than qualitative.15–17 Most recently, several new markers have been proposed that appear to be more lymphatic specific, particularly antibodies to lymphatic endothelial hyaluronan receptor-1 (LYVE-1; a homologue of CD44 glycoprotein),18 podoplanin,19 PROX1,9 and to a lesser degree, VEGFR-3 (the endothelial receptor for VEGF-C, the so-called lymphatic growth factor),20,21 as well as 5′-nucleotidase (see under the next section, Lymphatic-Specific Markers).22
Lymphatic-Specific Markers
Lymphatic vessels can increasingly be distinguished from blood vessels in tissue sections by whole-mount staining with specific markers. Only 10 years ago, little histochemical specificity existed to distinguish lymphatic vessels from blood capillaries and veins, and identification was based primarily on morphology, distinctive ultrastructure, or both. One of the most commonly used and most specific markers in use today is LYVE-1.19,23 It has been applied to tissues ranging from early mouse embryo to adult human and highlights collecting vessels and lymphatic capillaries (but not larger caliber vessels). Another strong marker localizing to the nucleus of lymphatic endothelial cells and adaptable to multiple tissues is the transcription factor PROX1.23 Podoplanin recognizes a transmembrane glycoprotein24 in lymphatics, but not blood vessel endothelial cells in the mouse, whereas its human analogue, D2-40,25 also sharply distinguishes lymphatic from blood vessel endothelium, but stains other distinguishable cells. This feature has been particularly useful in identifying preexisting and new lymphatics in tumor specimens and in generating quantitative differentials from blood vessels and indices of lymphatic invasiveness and tumor dissemination.26 5′-Nucleotidase staining is used by some research laboratories for its lymphatic specificity,22,27 and other common markers that show some cross reactivity to veins include VEGFR-310 and neuropilin-228 (reviewed elsewhere29,30). Thus, an array of lymphatic markers are now available to distinguish lymphatic from blood vessels, although there is some overlap of cell types in normal conditions and even more so in pathologic states.
Ultrastructure
Ultrastructurally, lymph capillaries display both “open” and “closed (tight)” endothelial junctions, often with prominent convolutions.31 Depending on the extent of tissue “activity,” these capillaries can dramatically adjust their shape and lumen size. Unlike blood capillaries, a basal lamina (basement membrane) is tenuous or lacking altogether in lymph capillaries.31,32 Moreover, complex elastic fibrils, termed anchoring filaments, tether the outer portions of the endothelium to a fibrous gel matrix in the interstitium.33–35 These filaments allow the lymph microvessels to open wide, which causes a sudden increase in tissue fluid load and pressure, in contrast to the simultaneous collapse of adjacent blood capillaries (Fig. 13-4). Just beyond the lymph capillaries are the terminal lymphatics. In contrast to more proximal and larger lymph collectors and trunks, the terminal lymphatics are devoid of smooth muscle, although the endothelial lining is rich in the contractile protein actin.15 Intraluminal bicuspid valves are also prominent features and serve to partition the lymphatic vessels into discrete contractile segments termed lymphangions.36 These specialized microscopic features of the lymphatic network support this delicate apparatus’ function of absorbing and transporting lymph node elements and the large protein moieties, cells, and foreign agents of the bloodstream (e.g., viruses, bacteria) that gain access to the interstitial space (Figs. 13-5 and 13-6).
Figure 13-4 A, Lymphatic capillary reconstructed from collated electron micrographs. The lymphatic anchoring filaments originate from the abluminal surface of the endothelial cells and extend into adjacent collagen bundles, thereby forming a firm connection between the lymphatic capillary wall and the surrounding interstitium. B, Transmission electron micrograph demonstrating anchoring filaments (AF) that derive from the lymphatic endothelium (LE) and join nearby collagen bundles (CB). C, Response of lymphatic capillaries to an increase in interstitial fluid volume. As the tissue matrix expands, tension on the AF rises, and the lymph capillaries open widely to allow more rapid entry of liquid and solute (a to c). In contrast to stretching of the lymph capillaries, a rise in matrix pressure collapses the blood capillaries, thereby restricting further plasma filtration. (From Leak LV, et al: Ultrastructural studies on the lymphatic anchoring filaments. J Cell Biol 30:129-149, 1968; and Leak LV: Electron microscopic observation on lymphatic capillaries and the structural components of the connective tissue–lymph interface. Microvasc Res 2:361-391, 1970.)
Figure 13-5 The lymphatic system consists of an initial superficial valveless network of endothelium-lined vessels connected to a deep valved collector system (lymphangion pumping segments defined by the valves). The deeper vessels run alongside major blood vessels and drain through lymph nodes to the main collectors; they ultimately empty into the thoracic duct or the right lymphatic duct. BK, Blood capillary network; C, cutis; CA, capsule; D, dermis; E, epidermis; F, fascia; M, musculature; MS, muscle layer; RS, marginal sinus; S, subcutis; SF, second follicle; SP, subcutaneous pseudofascia; V, lymphatic valves. (Modified with permission from BSN-Jobst Emmerich Conception, 2002.)
Figure 13-6 The postulated role of endothelial processes in microcirculatory events that have a bearing on angiogenesis in the blood-lymph loop. Such processes include macromolecular and liquid permeability, vasoreponsiveness, leukocyte adhesion and transmigration, coagulation cascading, particulate phagocytosis, antigen presentation and cytokine activation, lymphoid cell trafficking, and proliferative events leading to new vessel or tumor growth. Many mediators of these events have been identified by studying processes implicated at the blood vascular endothelial surface, which are likely to also occur at the lymphatic endothelial interface. The relative anatomic and dynamic relationships between blood and lymph vascular endothelium, parenchymal and extravascular connective tissue, and transmigrating leukocytes are shown. Black circle, exogenous particulates; black square, macromolecules; open circles, fluid (plasma, interstitial, or lymph). Ab, Antibody; BM, basement membrane; ECM, extracellular matrix; macro, macrophage; PMN, polymorphonuclear neutrophil; RBC, red blood cell; RES, reticuloendothelial system. (Modified from Witte MH, et al: Overlapping biomarkers, pathways, processes and syndromes in lymphatic development, growth, and neoplasa. Clin Exp Metastasis 29:707-727, 2012.)
Structural-Functional Imaging
Early physicians were able to visualize the lymphatic system only by observing chyle-filled mesenteric lymphatics. Asellius’ initial publication37 included what has been reported as the first color anatomic plate in history (Fig. 13-7).1 This was followed by intralymphatic injection of mercury into cadavers by Nuck in 1692, which depicted fine channels,38 then the detailed and elegant work of Mascagni in 1787,39 and subsequently, the classic images of both subcutaneous and deep vessels by Sappey in 1874.40 von Recklinghausen used silver nitrate, which allowed imaging to take place without removal of surrounding tissue and facilitated visualization of lymphatic vessels as distinct from blood capillaries.41 Gerota developed a technique in 1896 of injecting a mixture of Prussian blue and turpentine to highlight the vessels,42 and this was followed in 1933 by the intracutaneous injection of vital dyes that bind to tissue proteins by Hudack and McMaster,43 which is a technique still used today for investigation and in the clinic (Fig. 13-8).
Figure 13-7 Historical reproduction of Figure 1 from Gaspar Asellius (Gasparis Asellii) demonstrating chyle-filled lymphatic vessels of the mesentery. It is reported that this represents the first color anatomic figure ever published.1 (From a 1972 reproduction by Episteme Editrice, Milano, of the original text from Asellius G: De Lactibus sive Lacteis Venis Quarto Vasorum Mesaraicorum Genere Novo Inuento, Mediolani, 1627, JB Biddellium.)
Figure 13-8 Imaging techniques to delineate the structure and function of the lymphatic system. A, Evans blue dye injected intradermally in the tip of a mouse ear rapidly displays the draining lymphatic channels. A similar vital dye (lymphazurin blue or isosulfan blue) is used in the clinic. B, Classic lymphography image from Kinmonth clearly depicting the fine lymphatic vessels of the upper part of the thigh in an adult human.44 C, Radioisotope lymphangioscintigraphy displaying normal lymphatic tracer transport in the arms (upper panel) and the legs (lower panel). A single injection into each hand or foot is seen at the bottom of each image, with markers at the knees in the lower panel. (Modified from Witte CL, et al: Advances in imaging of lymph flow disorders. Radiographics 20:1697-1719, 2000.)
Modern imaging techniques also include direct (intralymphatic) injection of oily contrast agents, termed lymphangiography, as first described by Kinmonth in 1954,44 and whole-body lymphangioscintigraphy after subcutaneous or intradermal injection of protein-bound radiotracers (see Witte et al45 for an overview) (see Fig 13-8). Other agents used for indirect lymphography include a variety of fluorescent or magnetic particles,46,47 infrared particles and dyes,48–50 immunoglobulin conjugates,51 and microbubbles52 for detection with fluorescent microscopes, optical imaging systems, computed tomography (CT), magnetic resonance imaging (MRI) (with and without contrast),53 and ultrasound,54,55 with an expanding potential for highly specific molecular imaging. New contrast agents and techniques are continuing to be developed.56–58
Physiology
Any proteid which leaves these vessels…is lost for the time to the vascular system…it must be collected by lymphatics and restored to the vascular system by way of the thoracic or right lymphatic duct.
PHYSIOLOGIST ERNEST STARLING, 1897
General Principles
As a fine adjuster of the tissue microenvironment, the lymphatic system is often neglected in most treatises on vascular diseases. Yet this delicate system, so inconspicuous during life and collapsed after death, helps maintain the liquid, protein, and osmotic equilibrium around cells and aids in absorption and distribution of nutrients, disposal of wastes, and exchange of oxygen and carbon dioxide in the local milieu intérieur.
Interstitial (Lymph) Fluid
Two thirds of the body is composed of water, and most of this liquid volume is contained within cells. It is the remainder that exists outside cells, however, that continuously circulates. In a series of epochal experiments conducted more than a century ago, the English physiologist Ernest Starling outlined the pivotal factors that regulate partitioning of the extracellular fluid.59,60 In brief, the distribution of fluid between the blood vascular compartment and tissues and the net flux of plasma escaping from the bloodstream depends primarily on the transcapillary balance of hydrostatic and protein osmotic pressure gradients as modified by the character (i.e., hydraulic conductance) of the filtering microvascular surface (Fig. 13-9; also see Box 13-1). Normally, a small excess of tissue fluid forms continuously (net capillary filtration), and this surplus enters the lymphatic system and returns to the venous system. In contrast to blood, which flows in a circular pattern at several liters per minute, lymph flows entirely in one direction and at rest at a rate of only 1.5 to 2.5 L/24 hr. This limited volume derives from a slight hydrodynamic imbalance that favors movement of fluid, salt, and macromolecules from plasma into tissue spaces. Although blood capillary beds vary in hydraulic conductance, in general, disturbances in the transcapillary hydrostatic and protein osmotic pressure gradients (Starling forces) tend to promote edema that is low in protein content (<1.0 g/dL [10 g/L]), whereas impedance to lymph flow (lymph stasis) promotes (lymph)edema that is high in protein content (>1.5 g/dL [15 g/L]).
Figure 13-9 Primary forces regulating fluid flux into the interstitium and the importance of lymph flow in maintaining steady-state interstitial fluid volume, and hence, stable partitioning of extracellular fluid between the bloodstream and the interstitium (Starling’s equilibrium). Edema may occur as a result of high-output failure of lymph circulation (lymph overload with increased lymph flow) (lower left) or, less commonly, low-output failure (lymphedema) caused by interruption in lymph transport capacity (lower right).
Unlike blood flow, which is propelled by a powerful and highly specialized muscular pump (the heart), propulsion of lymph originates predominantly from spontaneous intrinsic segmental contractions of larger and probably also small lymph trunks (Fig. 13-10),61–63 and to a lesser extent, from extrinsic “haphazard forces” such as breathing, sighing, yawning, muscular squeezing (e.g., alimentary peristalsis), and transmitted arterial pulsations (Table 13-2).63,64 As noted, the contractions of lymphatic segments between intraluminal valves (i.e., the lymphangions) are highly responsive to lymph volume. Thus, an increase in lymph formation is accompanied by more frequent and more powerful lymphangion contractions (Fig. 13-11), a lymphodynamic response that resembles Starling’s other major physiologic principle, the law of the heart.36,65
Figure 13-10 Lateral pressure (blue curve) and cumulative lymph flow (red curve) in a subcutaneous leg lymphatic of a healthy man lying supine during movement of the foot and at rest. Note that lymph flow occurs only during rhythmic contraction of the lymphatic collector and specifically not by voluntary contraction of calf muscles. (Redrawn from Olszewski WL, et al: Intrinsic contractility of prenodal lymph vessels and lymph flow in the human leg. Am J Physiol 239:H775-H783, 1980.)
Figure 13-11 A human leg lymphatic has been cannulated retrograde, and the external tip of the cannula has been progressively raised to gradually increase hydrostatic pressure above the level of the cannulated lymphatic. A, While the man is upright, sequential increases in outflow resistance cause an increase in lymphatic pulse frequency (green curve) with subsequent decreases in pulse amplitude, lymph flow (blue curve), and stroke volume. When intralymphatic pressure reaches 34 mm Hg, lymph flow ceases despite a high pulsation rate. B, The same man is now tiptoeing, and rising outflow pressure induces more frequent lymphatic pulsations. Note again that calf muscle contraction with up-and-down foot motion is not associated with greater lymph propulsion. Also note that at higher pressures, each flow wave is followed by sporadic retrograde flow (reflux), which probably relates to intraluminal valve incompetence in the distending lymph vessel. (Redrawn with permission from Olszewski WL: Lymph pressure and flow in limbs. In Olszewski WL, editor: Lymph stasis: pathophysiology, diagnosis, and treatment, Boca Raton, FL, 1991, CRC Press, pp 136-137.)
Lymphatic truncal contraction, like venous and arterial vasomotion, is mediated by sympathomimetic agents (both α- and β-adrenergic agonists),66,67 by-products of arachidonic acid metabolism (thromboxanes and prostaglandins),68–71 and neurogenic, even pacemaker stimuli (Fig. 13-12).72,73 Oddly, in different regions of the body, lymphatic trunks seem to exhibit varying sensitivity to different vasoactive and neurogenic stimulants.67,74,75 Although the importance of truncal vasomotion as mediated by tunica smooth muscle is well established, it remains unclear whether terminal lymphatics or lymphatic capillaries are also capable of vasomotion or are simply passive channels. In some ways, this controversy parallels the prolonged dispute about whether blood capillary endothelial cells are capable of vasoactivity, an issue now clearly resolved in the affirmative. Because lymphatic endothelium, like blood endothelium, is rich in actin15 (a principal contractile protein), it is reasonable to assume that lymphatic microvessels also exhibit vasomotion.
Figure 13-12 Tracing taken from a human lower leg lymphatic segment. Norepinephrine (noradrenaline, NA), 10−6 M, induces phasic lymphatic contractions that are unaffected by the β-blocker propranolol, 10−6 M, but are abolished by phentolamine, 10−6 M. K+, Previous potassium (124 mM)-induced contraction; w, washout with fresh Krebs buffer solution. (Redrawn from Sjöberg T, et al: Contractile properties of lymphatics from the human lower leg. Lymphology 24:16-21, 1991.)
Lymph Nodes
In addition to their central immunologic role, lymph nodes are a potential site of impediment to the free flow of lymph. Unlike frogs, which lack lymph nodes but possess four or more strategically placed lymph hearts that propel large quantities of peripheral lymph back to the bloodstream,76,77 mammals possess immunoreactive lymph nodes, which when swollen, fibrotic, or atrophic, may act to initiate or perpetuate lymph stasis.78,79 Perhaps the intrinsic contraction of mammalian lymphatic trunks represents a phylogenetic vestige of amphibian lymph hearts (see the following section on Flow-Pressure Dynamics for more details).
Flow-Pressure Dynamics
Although lymphatic vessels, like veins, are thin-walled flexible conduits that return liquid to the heart, the flow-pressure relationships in the venous system and the lymphatic system are different. The energy to drive blood in the venous system derives primarily from the thrust of the heart. The cardiac propulsive boost maintains a pressure head through the arteries and blood capillaries into the veins. There is extensive neural regulation of venous tone that is essential for many activities, postural changes, and the negative pressure in the chest during inspiration. In addition, external compressive forces such as muscle can substantially alter venous pressure and gradients. Thus, muscular contractions such as walking and running, in the presence of competent venous valves, supplement cardiac action in facilitating return of blood to the heart.
In contrast, lymph vessels in tissues are not directly contiguous with the blood vasculature, and the chief source of energy for propulsion of lymph emanates from the intrinsic lymphatic truncal wall contractions (propulsor lymphaticum).61–77,80 Like amphibian lymph hearts (cor lymphaticum), mammalian lymphatic smooth muscle beats rhythmically, and in the presence of a well-developed intraluminal valve system, facilitates transport of lymph.81 In a sense, the lymphatic structures function as micropumps that respond to fluid challenges with increases in both rate and stroke volume.36,68 Ordinarily, resistance to flow in the lymphatic vessels is relatively high in comparison to the low resistance in the venous system,82 but the pumping capacity of the lymphatics is able to overcome this impedance by generating intraluminal pressures of 30 to 50 mm Hg (see Fig. 13-11) and sometimes even equaling or exceeding arterial pressure.61,82,83 This formidable lymphatic ejection force is modulated not only by filling pressure but also by temperature, sympathomimetics, neurogenic stimuli, circulating hormones, and locally released paracrine and autocrine cytokine secretions.84
It is often mistakenly thought that lymph return, like venous return, is directly enhanced by truncal compression from skeletal muscle and other adjacent structures. Although muscular contraction and external massage clearly accelerate lymph return in the presence of edema,83 under normal conditions, peripheral lymph flow is regulated primarily by spontaneous contraction of the lymphatics themselves.83,84 In peripheral lymphatics, unlike peripheral veins, the column of liquid is incomplete. Accordingly, with normal intralymphatic pressure, external compression is ineffective in propelling lymph onward (Fig. 13-13), although it may increase the frequency and amplitude of lymphatic contractions. In other words, lymphatics, in contrast to veins, are not sufficiently “primed.”85 During lymphatic obstruction and persistent lymph stasis, hydrostatic pressure in the draining tissue watersheds and lymphatics rise as intrinsic truncal contractions fail to expel lymph completely. In this circumstance, in contrast to the normal situation, the fluid column in the lymphatics becomes continuous, and skeletal muscle or forceful external compression then becomes an effective pumping mechanism that aids lymph transport.83,85
Figure 13-13 Effect of intermittent external pneumatic compression of the sheep foot on peripheral lymph flow, lymph outflow pressure, and venous pressure. Note that before external compression, the lymphatics contract spontaneously, and each contraction extrudes a small quantity of lymph, which appears as a step increment in the flow record. During the 2-minute period when the foot cuff is inflated intermittently, each compression causes a synchronous rise in peripheral venous pressure but not in lymph pressure. The frequency and amplitude of spontaneous lymphatic contractions increase, however, and this increase is associated with a concomitant rise in lymph flow. (Redrawn from Pippard C, et al: Comparison of fluid transport systems in lymphatics and veins. Lymphology 20:224-229, 1987.)
Studies of the effect of gravity on peripheral lymphatic and venous pressure confirm these findings. Although assuming an erect position sharply raises distal venous pressure, peripheral intralymphatic pressure is unaffected, although lymphatic truncal pulsation increases in both frequency and amplitude.85 This arrangement favors removal of tissue fluid by the lymphatics during dependency, because unlike veins, the lymphatics operate at much lower hydrostatic pressure. Once the lymphatics become obstructed, truncal contractions quicken at first, but then the intraluminal valves gradually give way, and as the lymph fluid column becomes continuous, this mechanical advantage is lost, and chronic lymphedema supervenes (Fig. 13-14).
Figure 13-14 The pathogenesis of peripheral lymphedema and some of its sequelae. According to this scheme, congenitally deficient or obstructed lymphatics promote lymph stasis, which is accompanied by deranged truncal contractility, progressive valve incompetence, destruction of contractile elements (lymphangioparalysis), and gradual ectasia of lymphatic collectors. After a variable period (occult lymphedema), sometimes aggravated by environmental trauma, a series of events is set into motion that culminates in chronic lymphedema. This clinical state is characterized not only by progressive swelling but also by fat and scar deposition, immunodysregulation, a propensity for cellulitis, and microvascular proliferation; these processes are essential for repair and regeneration but may result in bizarre and poorly understood new vascular growths.
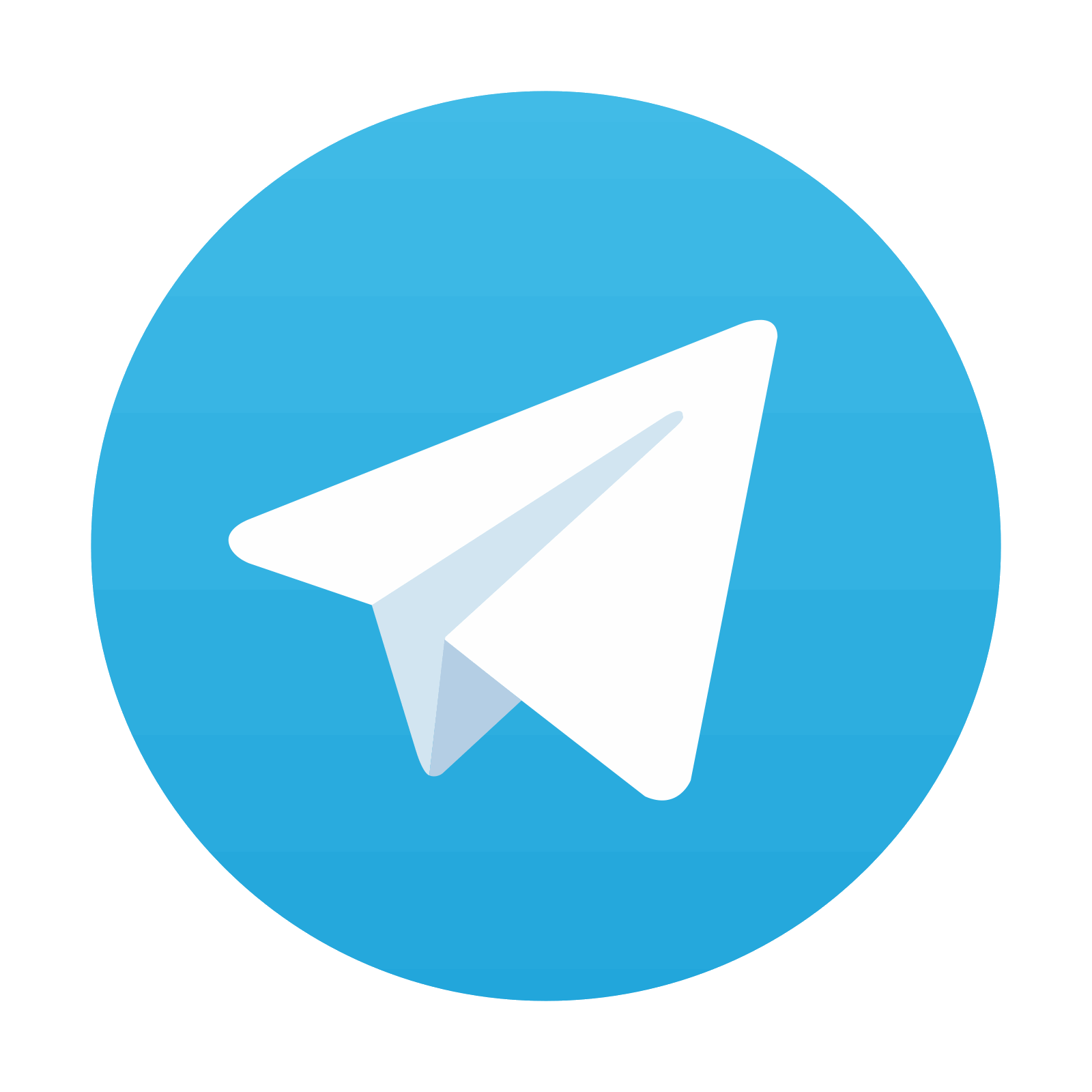
Stay updated, free articles. Join our Telegram channel
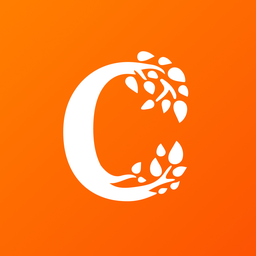
Full access? Get Clinical Tree
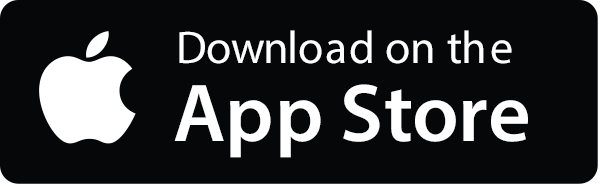
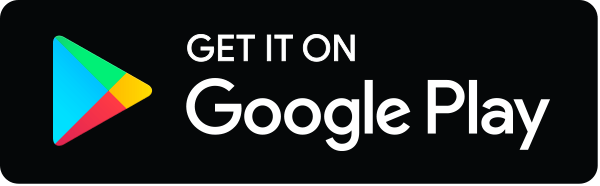