© Springer International Publishing Switzerland 2015
Ivan Bertoncello (ed.)Stem Cells in the LungStem Cell Biology and Regenerative Medicine10.1007/978-3-319-21082-7_33. Lung Vasculogenesis and Angiogenesis
(1)
Division of Neonatology, Department of Pediatrics, Sinclair Centre for Regenerative Medicine, Ottawa Hospital Research Institute, Children’s Hospital of Eastern Ontario, University of Ottawa, 501 Smyth Road, Ottawa, ON, Canada, K1H 8L6
(2)
Department of Pediatrics, Wells Center for Pediatric Research, Indiana University School of Medicine, Indianapolis, IN, USA
Keywords
AngiogenesisVasculogenesisLungDevelopmentInjuryAgingOxygenIntroduction
In 1959, Liebow observed that the alveolar septae in centrilobular emphysema were remarkably thin and almost avascular [1]. He postulated that a reduction in the blood supply of the small precapillary blood vessels might induce the disappearance of alveolar septae. Despite this early observation, pulmonary vessels were thought for many years, to be passive bystanders in lung development, following the branching pattern of the airways. At the other extreme of life, interrupted alveolar development in bronchopulmonary dysplasia (BPD), the chronic lung disease of prematurity, is also accompanied by a marked rarefaction of lung capillaries, suggesting a potential role of the developing lung vascular bed during normal alveolar development, injury, and repair. This review will summarize literature that has challenged old notions that the development of the blood vessels in the lung passively follows that of the airways. Evidence is presented that lung blood vessels actively promote alveolar growth during development and contribute to the maintenance of alveolar structures throughout postnatal life, opening new therapeutic avenues for lung diseases characterized by alveolar damage by modulation of vasculogenesis and angiogenesis.
Normal Lung Development in Brief
The classical stages of lung development: Lung development is classically subdivided into five overlapping stages in human and rodents, on the basis of gross histological features. The first four stages, termed the embryonic, pseudoglandular, canalicular, and saccular stages, occur during gestation. At the end of the saccular stage at about 36 weeks, lungs have formed alveolar ducts and air sacs. Alveolarization, the final stage of lung development, begins in the near-term lung prior to birth but primarily occurs postnatally, during the first 2–3 years of life, and may continue at a slower rate beyond childhood [2, 3]. The formation of alveoli occurs by the outgrowth of secondary septae that subdivide terminal saccules into anatomic alveoli. Premature infants at greatest risk for BPD in the post-surfactant era are born at 24–28 weeks, during the late canalicular or saccular stage of lung development just as the airways become juxtaposed to pulmonary vessels.
Lung angiogenesis: Formation of the pulmonary circulation has been primarily described as dependent on two basic processes: vasculogenesis and angiogenesis. Vasculogenesis is the de novo formation of blood vessels from angioblasts or endothelial precursor cells that migrate and differentiate in response to local cues to form vascular tubes. Angiogenesis is the formation of new blood vessels from preexisting ones. It has generally been accepted that the distal vasculature arises by vasculogenesis, and the proximal vasculature by angiogenesis, but this remains controversial [4]. De novo formation of blood vessels, i.e., vasculogenesis, from blood islands present within the mesenchyme have been described in the embryonic lung at embryonic day 9 (E9) in the mouse [5]. Angiogenesis starts around E12 when arteries and veins begin to sprout from the central pulmonary vascular trunks. Around E14, peripheral sinusoids and central vessel sprouts connect and establish a vascular network. This union of peripheral and central vascular structures is accompanied by extensive branching of the arteries, which follow the branching pattern of the airways. Again, the relative contributions of vasculogenesis and angiogenesis to lung vascular growth during each stage of lung development are controversial, and additional studies with appropriate experimental models are required to better define these underlying mechanisms. Studies in the developing chick embryo suggest that vasculogenesis is the main process by which the pulmonary vasculature forms [6]. Vascular fillings show that the lumen of the pulmonary arteries sprouted from the sixth pharyngeal arch arteries. However, serial sections and immunohistochemical localization of fetal liver kinase-1 protein (Flk-1), the receptor for vascular endothelial growth factor (VEGF) showed that the pulmonary arterial tree formed from endothelial cell precursors and coalescence of isolated blood vessels in the mediastinal splanchnic mesenchyme centrally to the developing lung tissue distally. Pulmonary veins grew from the left atrium to the developing lungs. Parera et al. suggested distal angiogenesis as a new mechanism for lung vascular morphogenesis, based on morphological analysis from the onset of lung development (E9.5) until the pseudoglandular stage (E13.5) in Tie2-LacZ transgenic mice [4]. In their model, capillary networks surrounding the terminal buds exist from the first morphological sign of lung development and then expand by formation of new capillaries from preexisting vessels as the lung bud grows [4]. The capacity of the primitive lung mesenchyme to differentiate into vascular endothelium was recently demonstrated in a new culture system and cells with the highest Flk-1 expression differentiated into endothelia more efficiently [7]. Studies in human fetal lung suggest that airways act as a template for pulmonary artery development, and that endothelial tubes form around the terminal buds of distal air spaces, suggesting an inductive influence of the epithelium [8]. Pereda et al. used immunolocalization and expression of CD31, CD34, FLT-1, KDR, and VEGF to show that at day 31 postfertilization (pf), a capillary plexus was already installed, and a few primitive erythroblasts were seen within the lumen of some blood vessels. Around day 45 pf, an increased amount of primitive erythroblasts was detected in the parenchyma surrounding the distal segment of the bronchial tree. The expression of FLT-1, KDR, CD31, and CD34 was observed in endothelial cells of the capillary plexus, and VEGF was detected in the endodermal epithelium. This indicated that the initial formation of the capillary plexus around the tip of the growing airway bud occurs by vasculogenesis likely regulated by VEGF. The increasing number of primitive erythroblasts from week 6 onward, associated with a change in the shape of the blood vessels, suggests a remodeling process and that the generation of new distal vessels at the tip of the lung bud occurs mainly by a process of angiogenesis. Clearly, new tools, such as lineage tracing studies routinely used in developmental biology, need to be applied to deepen our understanding about the formation of the pulmonary circulation [9].
The final important step of microvascular maturation overlaps the alveolar stage of lung development [10]. Capillaries, which are organized as double capillary layers in the immature gas-exchange region, later remodel to form a single capillary layer. This process is completed in the rat by about the third postnatal week. The alveolar wall thins and its cellular composition changes. In the rat, the thickness of the alveolar wall and the air–gas barrier (the distance between alveolar gas and capillary blood) decrease by 20–25 %. Between birth and adulthood, the alveolar and capillary surface areas expand nearly 20-fold and the capillary volume by 35-fold. Further expansion of the capillary network subsequently occurs via two angiogenic mechanisms: sprouting angiogenesis from preexisting vessels and intussusceptive growth [11]. Little is known about intussusceptive microvascular growth in the lung. This novel mode of blood vessel formation and remodeling occurs by internal division of the preexisting capillary plexus (insertion of transcapillary tissue pillars) without sprouting, which may underlie alveolar growth and remodeling throughout adult life and thus be amenable to therapeutic modulation for lung regeneration [12]. This is supported by recent findings suggesting that intussusceptive angiogenesis contributes to post-pneumonectomy lung growth. Much more needs to be learned about the anatomic events underlying lung vascular development and time-specific mechanisms that regulate growth and function at each stage. Nonetheless, current knowledge gained mostly from developmental biology and cancer literature about key angiogenic growth factors has been exploited to investigate the role of lung angiogenesis during normal alveolar development.
Evidence That Angiogenic Growth Factor-Driven Angiogenesis Promotes Normal Alveolar Development
VEGF is pivotal for the proper formation of blood vessels: VEGF is a highly specific mitogen and survival factor for vascular endothelial cells. VEGF binds to transmembrane tyrosine kinase receptors, VEGFR-1 (flt-1) and VEGFR-2 (flk-1/KDR), which are expressed on vascular endothelium [13]. The absolute requirement of VEGF for development of the embryonic vasculature in mice has been demonstrated by inactivation studies of VEGF alleles [14, 15] and knockouts of VEGFR-1 [16] and VEGFR-2 [17]. In each of these studies, inactivation of the target genes resulted in lethal phenotypes characterized by deficient organization of endothelial cells. Inducible Cre-loxP-mediated gene targeting or administration of a soluble VEGF receptor chimeric protein (mFlt (1-3)-IgG) to inactivate VEGF in early postnatal life results in increased mortality, stunted body growth, and impaired organ development [18]. VEGF inhibition resulted in less significant alterations as the animal matured, and the dependence on VEGF is lost around the fourth postnatal week. Interestingly, this period coincides with the end of alveolarization and microvasculature maturation in the lung.
VEGF promotes normal alveolar development: The spatial relationship between receptor and ligand suggests that VEGF plays a role in the development of the alveolar capillary bed. In addition, pharmacological and genetic inactivation of VEGF arrests alveolar development. VEGF mRNA and protein are localized to distal airway epithelial cells and the basement membrane subjacent to the airway epithelial cells [19]. This suggests that translocation of VEGF protein occurs after its synthesis in the epithelium. VEGFR-1 and VEGFR-2 mRNA expression also increases during normal mouse lung development [20, 21] and is localized to the pulmonary endothelial cells closely apposed to the developing epithelium [22]. VEGF120, VEGF164, and VEGF188 are present in alveolar type II cells in the developing mouse lung, and their expression peaks during the canalicular stage, when most of the vessel growth occurs in the lung, then decreases towards until day 10 postnatal (P10) when it increases to levels that are maintained through adulthood [22].
Targeted exon deletion of the VEGF gene reveals that mice that lack the heparin-binding isoforms VEGF164 and VEGF188 display a variety of vascular defects, including a significant reduction in the formation of air spaces and capillaries, resulting in distended and underdeveloped alveoli [23]. Likewise, pharmacological and genetic VEGF inhibition during alveolar development decreases alveolarization and pulmonary arterial density, features encountered in clinical BPD [23–26]. Chronic treatment of adult rats with the VEGFR-1 and -2 blocker SU5416 leads to enlargement of the air spaces, indicative of emphysema [27], suggesting that VEGF is required for the formation, but also the maintenance of the pulmonary vasculature and alveolar structures throughout adulthood. Conversely, lung overexpression of VEGF during normal lung development disrupts the lung architecture suggesting a tight regulation of VEGF-driven angiogenesis to insure proper lung development [28, 29]. Pharmacological VEGF inhibition in lung renal capsule grafts [26] and genetic VEGF inactivation [30] show that selective inactivation of VEGF in respiratory epithelium results in an almost complete absence of pulmonary capillaries, demonstrating the dependence of pulmonary capillary development on epithelium-derived Vegf-A. Deficient capillary formation in Vegf-A deficient lungs was associated with a defect in primary septae formation, coupled with suppression of epithelial cell proliferation and decreased hepatocyte growth factor (HGF) expression. Lung endothelial cells express HGF, and selective deletion of the HGF receptor gene in respiratory epithelium phenocopies the malformation of septae, confirming the requirement for epithelial HGF signaling in normal septae formation and suggesting that HGF serves as an endothelium-derived factor that signals to the epithelium. In summary, these observations suggest that inhibition of vascular growth itself may directly impair alveolarization.
Perturbation of nitric oxide (NO) is associated with arrested alveolar and lung vascular growth: While the role of the endothelium-derived relaxing factor NO in the regulation of the pulmonary vascular tone in the perinatal period is well established [31], little was known about its potential role in the structural development of the pulmonary vasculature. Studies suggest that VEGF-induced lung angiogenesis is in part mediated by NO. SU5416 (VEGF inhibitor)-induced arrested alveolar and vascular growth in newborn rats is associated with decreased lung eNOS protein expression and NO production; treatment with inhaled NO improves vascular and alveolar growth in this model [32]. Lungs of late fetal and neonatal eNOS-deficient mice have a paucity of distal arteries and reduced alveolarization [33] and are more susceptible for failed vascular and alveolar growth after exposure to mild hypoxia and hyperoxia [34].
Hypoxia inducible factors (HIFs): Despite its crucial role in O2 homeostasis, the role of HIFs during alveolar development was relatively unexplored until recently. HIF, a master regulator of O2 homeostasis discovered in 1992 by Dr. Semenza [35], regulates the expression of a range of angiogenic factors. HIFs are heterodimers that comprise one of three O2-sensitive α-subunits (HIF-1, HIF-2, or HIF-3) and a constitutively expressed β-subunit (also known as aryl hydrocarbon receptor nuclear translocator, ARNT) [36]. Under normoxic conditions, the α-subunit is rapidly hydroxylated by prolyl hydroxylase domain enzymes (PHD1-3) [37, 38] allowing recognition of the α-subunit by the von Hippel–Lindau (VHL) E3 ubiquitin ligase complex and subsequent proteosomal degradation [39].
Not surprisingly, HIFs contribute to normal lung development [40]. In the human fetal lung, all members of the HIF signaling pathway are expressed in the epithelium [41, 42]. HIF-2α and ARNT proteins are also localized in the mesenchyme [41, 42]. In vitro, HIF inhibition during early lung development, using antisense knockdown, decreases vascular development and lung branching morphogenesis in lung explants; exogenous VEGF only partially overcomes this arrest in lung development [43]. Conversely, conditional overexpression of HIF-1α in lung epithelium disrupts branching morphogenesis, impairs lung maturation, and causes pulmonary vascular abnormalities, including hemorrhage and increased lymphangiogenesis [44]. In contrast to the role of HIF during early lung development, there is relatively little information about HIF’s function during postnatal alveolar growth, mainly because HIF deficiency is embryonically [45, 46] or immediately postnatally [47, 48] lethal. Cre-mediated deletion of HIF-1α in the developing lung epithelium results in neonatal respiratory distress associated with decreased surfactant production [48]. HIF-2α−/− mice die at birth due to respiratory distress and can be rescued by VEGF treatment [47]. These observations suggest a role for HIFs during lung maturation and surfactant synthesis. The temporal expression pattern of HIF-1α mRNA and HIF-2α mRNA peaking during the alveolar stage of rat lung development provides circumstantial evidence for HIF’s role during postnatal lung development [49, 50]. HIF inhibition during the crucial period of alveolar development, by intratracheal dominant-negative adenovirus (dnHIF-1α)-mediated gene transfer or chetomin, a small molecule HIF inhibitor, efficiently decreased lung HIF-1α, HIF-2α, and VEGF expression and led to air space enlargement and arrested lung vascular growth [50].
PECAM–1: Administration of an anti-PECAM-1 antibody that inhibits endothelial cell migration, but not proliferation or survival in vitro, also impairs alveolarization in neonatal rats, without reducing endothelial cell content [51]. These data suggest that the loss of PECAM-1 function compromises postnatal lung development and provides evidence that inhibition of endothelial cell function, in contrast to loss of viable endothelial cells, inhibits alveolarization.
Evidence That Angiogenesis is Disrupted in Experimental Models of Arrested Alveolarization and in Human BPD and Emphysema
Link between angiogenesis and arrested alveolar growth/loss of alveoli: The proposed link between alveolarization and angiogenesis is suggested by the secondary abnormalities that occur in one process when the other is primarily affected. Alveolar hypoplasia and dysmorphic changes of the lung vasculature are consistent findings in experimental and human BPD. Already in 1959, Liebow had made similar observations in adult patients with centrilobular emphysema: alveolar septae were thin and nearly avascular, suggesting that a reduction in the blood supply of the small precapillary blood vessels might induce the disappearance of alveolar septae [1]. Lung vascular development is disrupted in all animal models mimicking BPD [52–55]. The first evidence that abnormal vascular development may contribute to neonatal lung disease came from autopsy studies showing reduced pulmonary microvascularization in infants dying from BPD [56]. A more recent postmortem study of newborns dying after short and prolonged durations of mechanical ventilation also quantified lung microvascular growth [57]. This study confirmed the reduction in vascular branching arteries, but interestingly lung PECAM-1 protein content (a marker of endothelial cells) was decreased in infants dying after brief ventilation, but was increased after prolonged ventilation [57]. These findings suggest a transient decrease in endothelial proliferation, followed by a brisk proliferative response, despite a reduction in vessel number. This observation suggests that dysmorphic lung vascular growth in BPD may not necessarily result simply from a reduction in the number of endothelial cells, emphasizing the need for more extensive studies in human and in animal models of BPD to better define the mechanisms that underlie early and the time-dependent sequence of events that precede the development of impaired distal lung structure.
Decreased VEGF and HIF signaling in BPD: Various animal models of impaired alveolar development also display abnormal lung vascular development [58–62]. Accordingly, animal and human studies show decreased expression of VEGF and its receptors in chronic newborn lung injury. Decreased VEGF expression is found in alveolar type II cells of newborn rabbits [63] or in newborn rat lungs [25] exposed to 100 % O2. In the preterm baboon model of BPD, arrested platelet endothelial cellular adhesion molecule-1 (PECAM-1, a distal lung endothelial cell marker) expression and reduced capillary density are associated with lower VEGF and VEGFR-1 mRNA and protein expression seen at 125 vs. 140 day term animals [64]. Similar observations exist in chronically ventilated premature sheep [65] and in term mice [66]. Premature sheep with antenatal endotoxin exposure (mimicking chorioamnionitis, another risk factor for BPD) also display decreased lung VEGF expression [67]. In humans, similar observations have been made in some [68–70], but not all [71] studies. Infants developing BPD have lower VEGF levels than those surviving without BPD [70]. VEGF may participate in pulmonary repair after acute lung injury. In lung tissue from infants who died from BPD, the typical patterns of alveolar simplification with “dysmorphic” microvasculature are associated with reduced lung VEGF and VEGFR-1 mRNA and protein expression [68]. Likewise, there are lower VEGF levels in the tracheal aspirates of preterm infants dying from severe respiratory distress syndrome than survivors and in infants subsequently developing BPD, as compared to premature infants surviving without pulmonary complications [68].
Hypoxia is a major stimulus of VEGF expression [72]. Premature exposure of the developing lung to a hyperoxic environment may downregulate VEGF expression. Even ambient O2 levels (21 %), i.e., premature birth per se, may interfere with normal lung vascular development [73]. Accordingly, HIF signaling is impaired in the lung of hyperoxic newborn rats [49, 50] and in large newborn animal models of ventilation-induced lung injury [74, 75] mimicking BPD.
Perturbation of other angiogenic growth factors provides further evidence for a link between disrupted angiogenesis and BPD: Despite its central role in vascular formation, VEGF works in concert with other factors, notably Angiopoietins (Ang) to stabilize the vascular wall. Unlike mouse embryos lacking VEGF or VEGFR-2, embryos lacking Ang1 or it receptor Tie2 develop a rather normal primary vasculature [76–78]. However, endothelial cells fail to associate appropriately with underlying support cells, which are the cells that provide the Ang1 protein that acts on endothelial Tie2 receptors [79]. While high concentrations of VEGF lead to immature, leaky, and hemorrhagic vessels, Ang1 generates vessels that are resistant to leak suggesting that Ang1 maximizes interactions between endothelial cells and their surrounding support cells and matrix [80]. These findings indicate that Ang1 is complementary to VEGF during vessel formation, acting at a later stage of angiogenesis to elicit vessel maturation and integrity. However, little is known about the role of Ang during normal alveolarization. In the baboon lung, Ang1 is mainly expressed in the septal mesenchymal cells, and Ang1 and its receptor Tie2 increase during lung development. Conversely, Tie-2 expression is decreased in lungs of ventilated baboons [64] and humans with BPD [81, 82]. The role of Ang2 during angiogenesis is less clear. Ang2 displays similarly high affinity for Tie2 and may act as a Tie2 antagonist. In lung epithelial cells, Ang2 expression is induced during hyperoxia [83]. Hyperoxia-induced oxidant injury, cell death, inflammation, permeability, and mortality are ameliorated in Ang2 knockout mice and in Ang2 siRNA-treated mice. Finally, Ang2 tracheal aspirate levels are increased in newborns that develop BPD. Likewise, chronically ventilated preterm lambs [84] and baboons [85] have decreased lung eNOS expression, suggesting that NO deficiency may contribute to the decreased alveolarization seen in these models of BPD.
Counter intuitively NF-κB, a transcription factor traditionally associated with inflammation, plays a unique protective role in the neonatal lung. Constitutive NF-κB expression is higher in neonatal lung and in primary pulmonary endothelial cells compared with the adult [86]. Inhibiting constitutive NF-κB activity in the neonatal pulmonary endothelial cells with either pharmacological inhibitors or RNA interference blocked pulmonary endothelial cells’ survival, decreased proliferation, and impaired in vitro angiogenesis. NF-κB was found to be a direct regulator of VEGFR2. Accordingly, blocking NF-κB activity during the alveolar stage of lung development in mice induced the alveolar simplification reminiscent of BPD and significantly reduced pulmonary capillary density. This data suggest that disruption of NF-κB signaling may contribute to the pathogenesis of BPD.
The Forkhead Box (Fox) family of transcription factors plays important roles in regulating expression of genes involved in cellular proliferation and differentiation. Newborn foxf1(+/−) mice with diminished Foxf1 levels displayed defects in alveolarization and lung capillaries and died postnatally [21]. Interestingly, surviving newborn foxf1(+/−) mice exhibited increased pulmonary Foxf1 levels and normal adult lung morphology, suggesting that wild-type Foxf1 levels are required for lung development and function. However, when challenged with butylated hydroxytoluene-induced lung injury, foxf1(+/−) mice died from severe lung hemorrhage and this was associated with decreased pulmonary Foxf1 expression and increased alveolar endothelial cell apoptosis that disrupted capillary integrity [87]. Thus, sustained expression of Foxf1 is essential for normal lung repair and endothelial cell survival. FOXF1’s crucial role in the formation of the vasculature was recently confirmed in mice with endothelial specific deletion of Foxf1 [88]. The clinical implication is that in humans, FOXF1 haploinsufficiency is associated with alveolar capillary dysplasia with misalignment of pulmonary veins, a rare, lethal developmental disorder of the lung [89].
Axonal guidance cues (AGC): are molecules that regulate neural network formation in the nervous system and the outgrowth of axons by acting as attractants, guiding neurons to their target or as repellents, creating exclusion zones that neurons avoid [90]. AGC are also involved in angiogenesis, cell migration, and early lung branching morphogenesis. Thus, AGC are appealing candidates in guiding also the outgrowth of secondary crests during alveolar development and repair [91]. Mice homozygous for the hypomorphic knockin allele ephrinB2ΔV/ΔV – encoding mutant ephrinB2 with a disrupted C-terminal PDZ interaction motif – die by 2 weeks of age and have enlarged air spaces, suggesting a crucial role for EphrinB2 during alveolar development [92]. EphrinB2 knockdown using intranasal siRNA during the postnatal stage of alveolar development in rats arrested alveolar and vascular growth. Conversely, hyperoxic exposed rats with enlarged air spaces and arrested lung vascular growth have decreased lung EphrinB2 and receptor EphB4 expression [93]. Similar observations have been obtained with semaphorins, another AGC family of molecules that play important neural and vascular roles [93–96].
Antiangiogenic factors: Endothelial monocyte activating polypeptide II (EMAP-II) and endostatin are potent natural angiogenesis inhibitors. The role of EMAP-II has been explored only at earlier stages of lung development, but its activation decreases neovascularization, interrupts lung branching morphogenesis, and decreases lung surfactant [97]. Endostatin was recently measured in tracheal aspirate fluid, and higher endostatin concentrations correlated with parameters reflecting lower lung maturity [98]. Endostatin may also contribute to aberrant epithelial repair in patients with pulmonary fibrosis that display elevated bronchoalveolar lavage endostatin levels [99]. These correlated with a number of elevated cytokines and impaired lung function in IPF.
It is very likely that other pro- and antiangiogenic growth factors play a role during lung angiogenesis and the balance between these growth factors is crucial for the coordinated assembly and remodeling of blood vessels during alveolar development. For example, hyperoxia increases the potent angiostatin, Pigment Epithelium-derived Factor (PEDF), in neonatal mice [100]. Conversely, hyperoxia reduced alveolarization in wilt-type mice, but not in PEDF (−/−) mice. Interestingly, VEGF stimulation of proliferation, migration, and capillary tube formation of MFLM-91U (a fetal mouse lung endothelial cell line) was inhibited by PEDF. MFLM-91U cells exposed to conditioned medium from E17 fetal mouse lung alveolar type II cells cultured in hyperoxia formed fewer capillary tubes than conditioned medium from type II cells cultured in room air. This effect was abolished by PEDF inhibition. These data support that interrupted alveolar development in BPD likely results from an altered balance between pro- and antiangiogenic factors.
Endoglin (CD105) is a hypoxia-inducible transforming growth factor-beta (TGF-β) co-receptor. Excessive lung TGF-β expression contributes to arrested alveolarization [101]. High levels of endoglin expression have been described in vascular endothelial cells in tissues undergoing active angiogenesis, such as in regenerating and inflamed tissues or tumors [102]. Lungs of short-term ventilated preterm infants showed significant upregulation of endoglin mRNA and protein levels, immunolocalized to the microvasculature compared to age-matched nonventilated control lungs [103]. Similar but more variable endoglin upregulation was noted in lungs of long-term ventilated infants with BPD. This was associated with decreased mRNA levels of VEGF, Ang1, and their respective receptors [103].
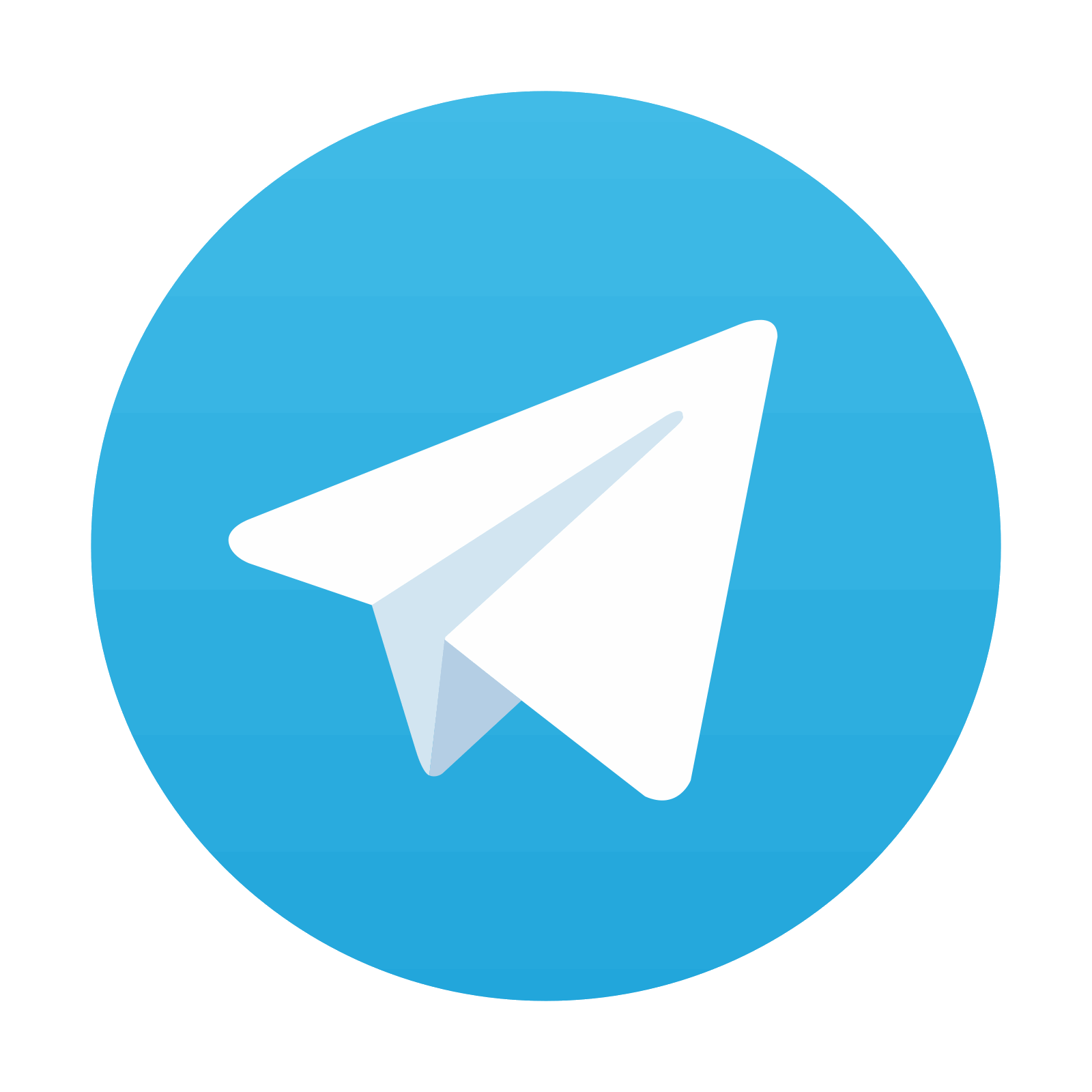
Stay updated, free articles. Join our Telegram channel
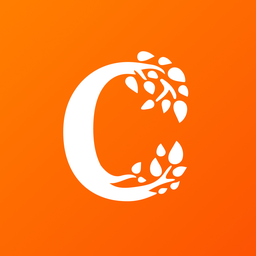
Full access? Get Clinical Tree
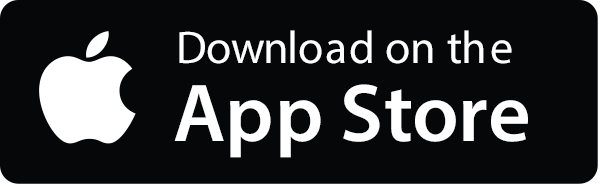
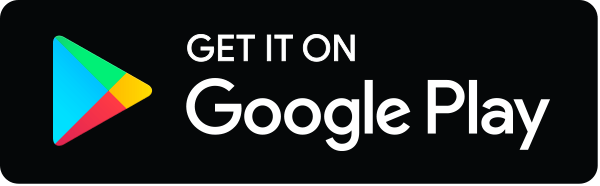