The stages of lung development and the development of the bronchial tree. On average an airway of a human lung ends after approximately 23 generations in an alveolar saccule; however, due to the shape of the lung, a range of 18 to 30 generations has been observed for lung pathways of varying lengths.
The Pulmonary Vascular Tree
Bronchial circulatory structures are also highly specialized, conforming to function (8). Elliott and Reid (9, 10) categorized pulmonary vessels into conventional and supernumerary; conventional vessels are located adjacent to the bronchial tree, and their branches and supernumerary vessels supply the nearest acinar structures. Supernumerary vessels are found throughout the pulmonary tree, but tend to be more numerous toward the periphery of lung tissue. Developmentally, the conventional and supernumerary vessels are established in the same temporal space as the pulmonary structures they accompany/supply, and pre-acinar pulmonary vessel anatomy is complete at midgestation. Vascular development after 20 weeks consists largely of changes in vessel wall thickness, cellular constituents, and muscle formation. At birth, pulmonary arterial walls quickly thin (by around 50% as smooth muscle cells reduce their overlap), and the medial thickness continues to decrease over the first 3 months of life, with little physiological change thereafter. The rate of arterial formation increases for 2 months after birth, and then slows to match the rate of alveolar formation. These anatomical changes reflect functional changes throughout late gestation and birth/perinatal development. Pulmonary circulation in the fetus has relatively high vascular resistance and low blood flow (11), which changes at birth and the first inspiration when the foramen ovale and ductus arteriosus close, shunting blood to the lungs (12). Higher PO2 in the pulmonary artery is sensed by the endothelium, and higher shear stress acts to switch the function of these vessels in the breathing infant (13).
Pre- and Postnatal Lung Development
Embryonic Stage (Weeks 4–7 p.c., 6–9 Weeks PMA)
Tree-like growth of the lung begins during the first few weeks postconception; the embryonic stage is characterized by branching morphogenesis of both the airways and blood vessels, arising from a mixture of largely undifferentiated cells that will later segment themselves into the smooth muscle, blood vessels, lymphatic cells, and epithelium. It was once thought that both lungs formed from a single anlage; however, we now know that each of the two lungs is formed by an independent anlage (14,15). The two lung buds first appear at day 26 p.c. (postcoitum, days after conception)/5 weeks PMA (postmenstrual age), having emerged from the ventral wall of the primitive foregut endoderm at approximately 3 weeks p.c./5 weeks PMA (Figure 7-2). The lung buds then grow into the surrounding mesoderm just anterior and parallel to the initial formation of the esophagus. On week 4 p.c./6 weeks PMA, when the embryo is still only a few millimeters long, the trachea (formed from the fusion of the lung buds) and larynx from just above the lung anlage. Between days 33 and 41 p.c. (week 7 PMA) the second bifurcation occurs, forming what will eventually be the lobes (two left and three right) of each of the lungs. A third round of branching occurs at week 8 PMA (41–44 days p.c.), with approximately 8–9 and 10 segmental branches on the left and right respectively, which establishes the bronchopulmonary segments of the mature lung.
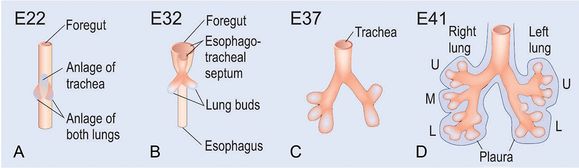
At day 26 postcoitum (p.c.) the anlage of the two lungs forms on both sides lateral of the anlage of the trachea by outpouchings of the foregut (A) (15). The prospective trachea forms by a distal-to-proximal segregation from the foregut. At day 32 the lung anlage gives rise to the future main bronchi (B). The lobar bronchi are formed due to continued branching at day 37 (C). Later at day 41 the segmental bronchi are following (D). After the formation of the pleura, organogenesis is completed (D). U, upper lobe; m, middle lobe; l, lower lobe
Significant proliferation of mesenchymal and epithelial cells occurs during this stage, with apoptosis near branch points and in the areas of new formation. The mesenchyme will later differentiate into progenitor cells, which will give rise to blood vessels, smooth muscle, cartilage, lymphatics, and connective tissue. At the embryonic stage, the epithelial cells are simple and undifferentiated, forming a pseudostratified columnar epithelium. The mesenchyme remains loosely constructed to allow mass flow of proteins necessary for development. The basement membrane, however, is intact and patent, which is an important control mechanism. Although the primitive trachea and esophagus are completely separated, each lack cartilage, smooth muscle, and nerves. By the end of this stage, the primitive vasculature has been established with pulmonary arteries branching from the embryonic sixth aortic arch and veins emerging from the left atrium of the developing heart.
Clinical Aspects of the Embryonic Stage
Perhaps unsurprisingly, abnormalities in this stage of lung development are critical in postnatal mortality and morbidity of the fetus and are often related to lung bud development, trachea/esophagus separation, proximal airway, and initial lobe formation. Some structural abnormalities such as pulmonary valve stenosis, pulmonary agenesis, or aplasia generally are considered nonsurvivable, whereas others may cause significant pulmonary morbidity. Tracheoesophageal fistula, which encompasses a variety of permutations of abnormal connections between the trachea and esophagus, is a common congenital abnormality associated with this stage of lung development, often accompanied by esophageal atresia, a defect whereby the upper and lower segments of the esophagus do not connect. Postsurgical survival in full-term infants is high, but in low-birth-weight infants, tracheoesophageal fistula is often accompanied by other congenital malformations and a concomitantly higher risk for poor outcomes (16).
Pseudoglandular Stage (Weeks 5–17 p.c., 7–19 Weeks PMA)
By week 6 p.c./week 8 PMA of development, the growing lungs can be distinguished as two separate organs, assuming a glandular appearance consisting of multiple, branching epithelial tubules surrounded by extensive regions of mesenchyme. Early in this stage of lung development, cellular proliferation increases, accelerating airway and vascular branching. By week 17 p.c./week 19 PMA), the conducting airways including the terminal bronchioles are formed – the upper lobes having 12–17 airway branching generations, 18–23 generations in the middle lobes, and 14–23 generations in the lower lobes.
At the beginning of the pseudoglandular stage, the respiratory bronchioles are lined with pseudostratified columnar epithelium, supported by a basement membrane scaffold with adjacent mesenchyme, all surrounded by an extracellular matrix rich in the macromolecules needed for cellular differentiation, migration, adhesion, and proliferation (Figure 7–3A, D). The respiratory epithelium differentiates, forming tall columnar epithelial cells in the proximal airways and cuboidal epithelium in the distal acinar tubules. Further differentiation forms ciliated and mucous cells in the trachea and proximal airways. Supportive cartilage appears in the trachea by week 10 p.c. The mesenchymal cells differentiate, forming myofibroblasts by week 7 PMA, thus laying the foundation for the developing smooth muscle layer to form around the tubules. By the end of the pseudoglandular stage (week 17 p.c.), cartilage extends to the segmental bronchi and smooth muscle extends to the alveolar ducts.
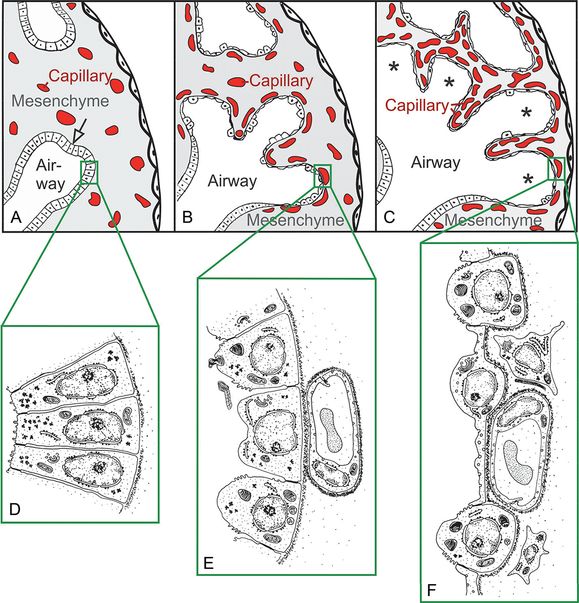
The epithelial tubules branch constantly during the pseudoglandular stage and penetrate into the surrounding mesenchyme (open arrow, branching point, (A). The mesenchyme contains a loose capillary network (A). The epithelium itself is tall and columnar (D). The canalicular stage (B) is characterized (1) by a differentiation of the tall columnar epithelial cells into prospective lining and secretory cells (type I and type II epithelial cells, E and F), (2) by a widening of the future airways (B), (3) by a multiplication of the capillaries and their first close contacts to the epithelium (B), and (4) by the formation of first air–blood barriers (E→F). Throughout the saccular stage (C) the mesenchyme further condenses, resulting in the formation of the thick immature inter-airspace septa, which contain a double-layered capillary network – one layer on either side of the septum. The widened terminal ends of the bronchial tree are recognized as saccules (asterisks).
Cell growth and differentiation drive the development of the pulmonary arteries and veins, and by the end of this stage, all pre-acinar vasculature is formed from endothelial tubes. In the distal branches of the terminal bronchioles, acinar tubules and buds establishing the primitive acinus are also formed by the end of the pseudoglandular stage (17).
Clinical Aspects of the Pseudoglandular Stage
In this stage, spontaneous, peristaltic contractions of the airway smooth muscle are observed. There is firm evidence to support that a maintained pressure differential between the airway lumen and surrounding tissue is essential for normal lung development. It is postulated that these contractions contribute to fetal lung development by mechanoreceptor-mediated growth factor production (18) and also function to prevent uncontrolled distension of the airways as lung fluid is secreted into the lumen (18).
It is also in this stage (around 10 weeks p.c.) that fetal breathing movements can be discerned (19). Recent in vitro studies have investigated the signaling pathways linking fetal breathing movements and lung development. These movements stretch and move fluid into and out of the lungs. Mechanical stretch upregulates the release of serotonin via mechanosensitive channels (20), which promotes differentiation of epithelial cells. Stretch also increases epithelial cell proliferation (21) and stimulates secretion of lung surfactant lipids from type II epithelial cells (22).
Developmental abnormalities during the pseudoglandular stage are generally related to airway and vascular branching and growth. Abnormal branching can result in pulmonary hypoplasia or sequestration. Absence or abnormal weakening of supportive cartilage is associated with tracheo- or bronchomalacia, which, respectively, can cause trachea or airway collapse during respiration. Importantly, during this stage of development, the pleuroperitoneal membrane closes. Failure of the membrane to close completely often results in the herniation of the developing abdominal organs into the chest cavity. This congenital diaphragmatic hernia (CDH) often leads to pulmonary hypoplasia and pulmonary hypertension, and although the underlying cause of CDH remains unknown, there is evidence to implicate genetic mutations to the transcriptional and growth factors related to lung development (23).
Canalicular Stage (Weeks 16–26 p.c., 18–27 Weeks PMA)
The canalicular stage is characterized by the differentiation of the epithelial cells, angiogenesis of the parenchymal capillary network, and the first formation of the air–blood barrier. Some of the most distal generations of airways (future alveolar ducts) still remain to be formed at this stage (17).
“Canalization” of the Lung Parenchyma. Before the first air–blood barriers can be formed the mesenchymal capillaries must be proximal to the epithelial cell lining of the future airways. Two steps are necessary for this process: (1) During the pseudoglandular stage the mesenchymal capillaries form a loose three-dimensional network. A high density of capillaries is achieved by an enhanced angiogenesis (Figure 7-3 A→B). The respiratory airways (airways distal of the purely conducting airways) start to grow in length and width; their shape changes, and they are also called “canaliculi.” This canalization of the respiratory airways and capillaries contributes to the nomenclature for this stage. (2) Due to increasing volume of the future airways, the mesenchyme condenses; as a result the epithelium of the future airways comes into close contact with the mesenchymal capillary network (Figure 7-3 A→B). Programmed cell death (apoptosis) occurs during condensation of the mesenchymal tissue. The involvement of apoptosis is consistent with a reduction of mesenchymal cells during this stage.
Epithelial Differentiation – Formation of the Air–Blood Barrier
During the canalicular stage, the differentiation of the parenchymal epithelium becomes morphologically visible, even if the cell fate is decided much earlier (15). The cuboidal glycogen-rich epithelium of the future gas-exchange region differentiates into the type I and type II alveolar epithelial cell (Figure 7-3 E→F). Type II alveolar epithelial cells are the progenitors of type I epithelial cells (24). Therefore, it is not surprising that the future alveolar epithelial cells contain few, small, lamellated bodies before a differentiation into type I and type II cell becomes morphologically visible (25).
The type I cells spread out and form thin sheet-like extensions covering most of the inner surface of the future gas-exchange region (Figure 7-4). At locations where the type I alveolar epithelial cells make close contact with the endothelium of the capillaries, an air–blood barrier forms (Figs. 7-3 b, e→f; 7-4 a). The tissue of the air–blood barrier is reduced to a sheet-like extension of the type I epithelial cell and the thin part of the endothelial cell. Both cell types are separated by their basement membranes, which are fused to one common basement membrane possessing one central lamina densa and two lamina lucida facing the epithelial or endothelial cells, respectively (Figures 7-3F, 7-4A, B). Currently, it is poorly understood how the air-blood barrier forms; it is likely that mesodermally derived endothelium interacts with endodermally derived epithelium (26). This hypothesis is supported by a phenotype of a transgenic mouse, where the sequence coding for the nitrogen-binding site of laminin (γ1III4, within the laminin-γ1-chain) was selectively deleted. The basement membranes of the air–blood barrier were disrupted or missing in large part in these mice. Due to a failure of pulmonary gas exchange the mice died at birth (27).
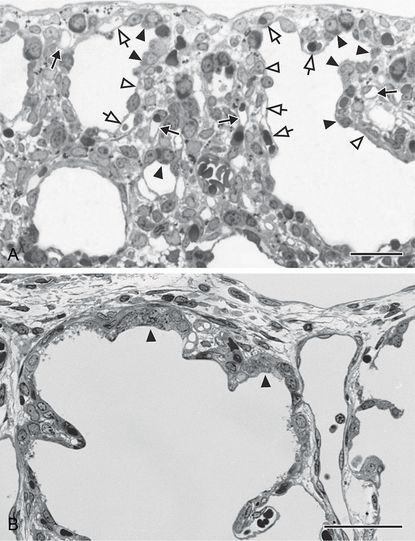
During the early canalicular stage (A, rat lung) the epithelium of the terminal airways is still cuboidal and glycogen-rich (closed arrow head). Already a bit more proximal, the epithelium begins to flatten out (open arrow head) and to form the first thin air–blood barriers. During the latter process capillaries, which are located inside the mesenchyme (closed arrow), “move” toward the epithelium (open arrow). In human lung (B, postnatal day 26) remnants of the cuboidal epithelium (closed arrow head) are still present at the uttermost periphery of the gas-exchange region – even if alveolarization started already (B). This demonstrates the large overlap between different phases of lung development, especially if peripheral and central parts are compared. In addition, it illustrates the central-to-peripheral progression of lung development. Light microscopical images, bar, 50 μm.
During the canalicular stage, type II epithelial cells start to produce surfactant, which is morphologically visible by the first accumulation of lamellar bodies. In most species surfactant appears late in gestation (at about 80–90% of the total duration of gestational period). In humans small amounts of surfactant are already present at weeks 22–24 p.c./weeks 24–26 PMA (60% gestation) (28), which facilitate survival of very prematurely born babies. Before the lung fully matures, surfactant appears to be more abundant in apical than in basal lung regions (29). This observation may explain clinical findings that in some prematurely born babies, hyaline membrane disease is more pronounced in basal than in apical lung regions.
An acinus is a small tree of gas-exchanging airways, which is fed by the most distal purely conducting airways. Boyden (30) observed the birth of the acinus during the canalicular stage; however, the airways are already formed during the pseudoglandular stage (17). Due to the aforementioned differentiation of the epithelia, a distinction of conducting and gas-exchanging airways becomes feasible during the canalicular stage, and therefore the acini may now be recognized.
Clinical Aspects of the Canalicular Stage
The canalicular stage may be the most critical stage for very immature, prematurely born infants. After the formation of the first air–blood barrier and the start of a minimal production of surfactant, some pulmonary gas exchange becomes possible. At the end of the canalicular stage, survival is feasible – but only with the specialized support of a neonatal intensive care unit. Survival itself is strongly dependent on the amount of functional gas-exchange surface area, which is determined by the amount of formed air–blood barrier and by the amount of available surfactant. Even if the surface area of the air–blood barrier is sufficient, only limited areas of the lung will be inflated if surfactant is not available in adequate amounts.
Alveolar capillary dysplasia (ACD, also congenital alveolar dysplasia) is a very rare congenital disease that is a malformation or absence of the pulmonary blood vessels, in particular of the alveolar capillaries. In principle, the blood vessels develop in parallel to the airways, and therefore, the etiology of ACD is already present at the pseudoglandular stage. The malformation becomes more pronounced in the canalicular stage because the surface area of the air–blood barrier is greatly reduced, concomitant with a greatly reduced capillary mesenchymal network. In addition to a reduction of pulmonary gas-exchange capacity, ACD causes persistent pulmonary hypertension.
Saccular Stage (Weeks 24–38 p.c., 26–40 Weeks PMA)
The saccular stage represents an intermediate stage between completion of branching morphogenesis and the start of the alveolarization. While the most distal generations of airways have likely been already laid down by the end of the canalicular stage, the addition of a very few terminal airways may still occur during the beginning of the saccular stage in some species (31). The name “saccular stage” describes the typical appearance of peripheral airway clusters forming widened airspaces called saccules or terminal sacs.
Expansion of the Gas-Exchange Area
Airspace generations distal to the terminal bronchioles are widening and lengthening during this stage and are forming the required space for subsequent alveolarization. At the terminal end of the bronchial tree, sacculi form (Figure 7.3D, F); these sacculi are smooth walled, separated by septa, and covered with type I and II pneumocytes. The primary septum contains two networks of capillaries for the adjacent sacculi, as well as some elastic and collagen fibers. At this stage, the interstitial space is still cell-rich and has an important regulatory and developmental role for the epithelium above (32). By term birth, all generations of the conducting and respiratory branches have been generated. The sacculi are thin, smooth-walled sacks and correspond to the later alveolar sacculi. At the end of this phase, the interstitial fibroblasts begin with the production of extracellular material in the interductal and intersaccular space.
Time of Birth. The maturity of the lung at birth of different species correlates with the physical activity of the newborns (Table 7-1). If high activity is required at birth, the lungs are near fully matured and capable of high amounts of gas exchange. For example, in precocial animals like sheep (33) and guinea pigs (34), alveolarization starts well before birth, and at term, their lungs appear nearly mature. Alveolarization of altricial species, such as rats (35), mice (36), and humans (37), starts only shortly before birth (humans) or after birth (mice and rats). At the extreme end of this range, one of the most immature lungs at birth is the lung of the marsupial quokka wallaby (Setonix brachyurus), which is born in the canalicular stage (38). Regardless of how mature the lung may be at term, alveolarization continues at least as long as the lungs are growing – until young adulthood in most species (14).
Because the stages are defined mainly by morphological criteria, their beginning and end do not represent sharp borders. In addition, stages may overlap (in particular the alveolar stages and the stage of microvascular maturation), and regional differences are also common – especially between central and peripheral regions. Nutrition and litter size influence the exact timing of development (28, 47, 69–72). Monkey, Rhesus monkey; E = embryonic day (days postcoitum); n.d., not determined; P, postnatal day; * weeks postcoitum; ** unpublished observation of JCS (based on Schittny and Burri 2008 (14)).
Clinical Aspects of the Saccular Stage
At this stage, imbalances in the pressure differential between intraluminal airway and extraluminal spaces can have lasting detrimental effects on lung development. Fetuses exposed to oligohydramnios have an 80% higher risk of respiratory failure than their unexposed counterparts (39). Oligohydramnios is associated with pulmonary hypoplasia and is often part of a pleiotropic manifestation of autosomal recessive polycystic kidney disease (40).
BPD (bronchopulmonary dysplasia, a chronic lung disease of infancy) is the most common cause of pulmonary morbidity in premature infants and is associated with long-term morbidities (41). BPD is a complication of respiratory distress syndrome of premature birth and is most often seen in very low- or extremely low- birth-weight infants who required ventilation, antenatal steroids, and surfactant therapy for survival. Infants with BPD typically have fewer, larger alveoli (and therefore decreased gas-exchange surface area) and insufficient vascularization to the alveoli (and therefore less-efficient gas exchange) than their term counterparts. A number of factors can contribute to BPD, including maternal drug use or smoking, extent of prematurity, sepsis, and overly aggressive ventilation. Recent studies postulate a role for stem cell depletion in the etiology of BPD, and stem cell therapy has proven beneficial in experimental models (42). Children who were preterm have a significant airflow limitation during the first years of life, and their lung function can deteriorate in their first year (43).
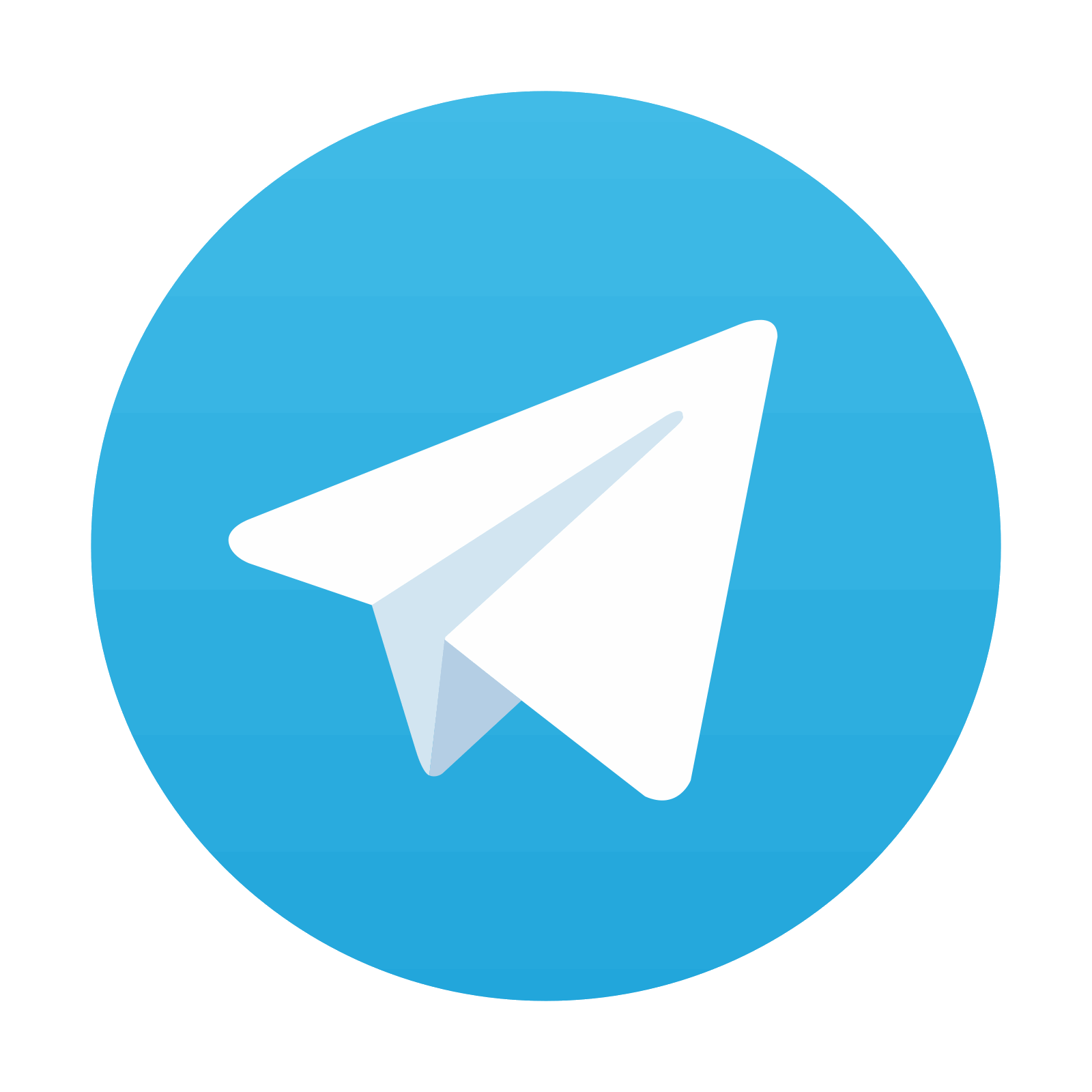
Stay updated, free articles. Join our Telegram channel
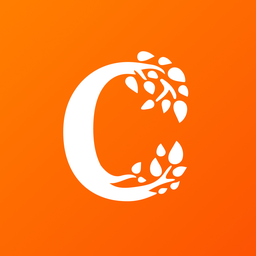
Full access? Get Clinical Tree
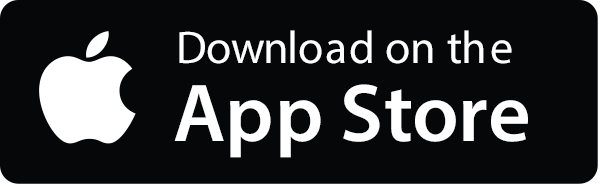
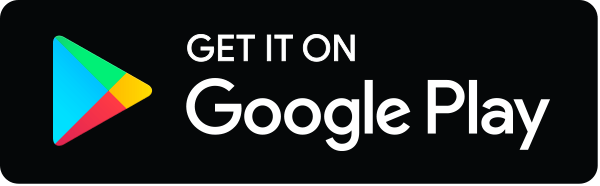