Introduction
Multicellular life requires the use of oxygen for the generation of high-energy compounds (e.g., adenosine triphosphate) to sustain the metabolic activities of complex organisms. Because multicellular organisms depend on oxygen, they have evolved systems for its efficient acquisition and distribution. A benchmark in the adaptation of vertebrates living on land was the development of a gas exchange system that provided a sufficient amount of oxygen to meet the metabolic requirements of cellular respiration. As organisms increased in size, the surface area required for adequate gas exchange became significantly larger; for example, the surface area of the adult human lung epithelium has been estimated to be 70 m 2 . The problem of generating such a large surface area in a confined space has been solved in the basic structure of the lung, where branched epithelial tubules conduct air to millions of alveoli that lie closely apposed to the lung microvasculature. The epithelium lining the surface of the lung is continuously exposed to biologic and chemical hazards from the environment, which has also necessitated the development of an innate defense system in the lung. Early embryologic experiments established that lung morphogenesis is critically dependent on reciprocal interactions between the lung endoderm and its surrounding splanchnic mesoderm, which supplies progenitors of endothelial cells, smooth muscle cells, mesothelial cells, and fibroblasts. As we will discuss, these interactions are complex and highly regulated in time and space. Disruptions in the lung developmental program, be it for genetic or epigenetic reasons, can lead to compromised structure and function. A better understanding of the molecular mechanisms controlling lung development will optimize therapeutic strategies to treat the diseased or malformed lung. Several additional recent reviews are also available.
Stages of Lung Development
Lung development has traditionally been divided into five stages that are primarily based on histologic appearance ( Fig. 2-1 ). After lung bud formation the basic branching pattern of the pulmonary tree and an associated vascular plexus is established during the embryonic and pseudoglandular stages. The epithelial branching program, which is under genetic control, is stereotyped and uses three geometrically distinct local modes of branching that proceed in three different sequences. Human lung development begins with the emergence of the laryngotracheal groove from the floor of the foregut endoderm during the fourth week of gestation. A few days later the caudal end of the primordium enlarges and bifurcates, giving rise to the left and right bronchial buds (see Fig. 2-1A ). These buds elongate caudally during the fifth week of gestation, when a second round of branching takes place, resulting in three secondary buds in the right lung and two in the left. These buds will become the primary lobes of the left and right lung. A third round of branching gives rise to bronchial tubules that will become the bronchopulmonary segments in the mature lung. Concurrent with these events in the distal region, the cranial portion of the primordium gives rise to the trachea and larynx, which separate from the esophagus by the end of this stage. The lung epithelium at this stage is tall columnar and shows no morphologic evidence of differentiation. At the molecular level, however, some aspects of epithelial differentiation have already begun; for example, the most distal epithelial cells express messenger RNA for the lung-specific marker surfactant protein (SP) C. The lung mesenchyme, which is derived from splanchnic mesoderm, is loosely organized at the beginning of this stage and appears to lack vascular structures. In situ hybridization studies probing for the vascular endothelial growth factor (VEGF) receptor FLK1 have demonstrated, however, that vascular precursors are closely apposed to the distal epithelium at the time of bud induction. These cells form a vascular plexus (see Fig. 2-1B ) by a process termed “vasculogenesis,” wherein vessels are formed de novo by the organization of vascular precursors. By the end of the embryonic stage, pulmonary arteries and veins connect this plexus to the atria; the pulmonary arteries and veins grow into the lung by angiogenesis, with new branches arising from preexisting vessels.






Dichotomous and lateral branching of the lung epithelium continues during the pseudoglandular stage, which lasts from week 5 to week 17 of gestation. This results in the final pattern of the pulmonary tree, which comprises 22 to 23 generations of bronchial tubules. Terminal bronchioles branch distally to give rise to the acinar tubules and buds that will eventually form pulmonary acini in the adult (see Fig. 2-1C ). Morphologic differences in the epithelium are apparent. The proximal epithelium is initially populated by relatively undifferentiated columnar, glycogen-rich cells, but ciliated, nonciliated, goblet, mucous, basal, and neuroendocrine cells are identifiable by the end of this stage. The distal epithelium is populated by distal epithelial cells, the precursors of alveolar type II cells, which are cuboidal columnar and contain copious amounts of glycogen. Smooth muscle cells differentiate in the mesenchyme and surround the epithelium perpendicular to the long axis of the tubules; this proceeds in a proximal-to-distal manner. The pulmonary vasculature branches in parallel with the airway epithelium (see Fig. 2-1C ), and pulmonary lymphatics initiate as buds from the veins.
Patterning of the pulmonary tree is completed at the beginning of the canalicular stage (16 weeks to 26 weeks; see Fig. 2-1D ), and the cells constituting the proximal epithelium continue to differentiate as ciliated, nonciliated, and secretory cells. Among the latter are club cells (Clara), identifiable by the presence of the cell-specific club cell secretory protein ( Clara ) (CCSP). Acinar tubules and buds, which are lined by cuboidal epithelial cells, expand and differentiate to form pulmonary acini consisting of respiratory bronchioles, alveolar ducts, and alveoli. Nascent type II cells containing increasing amounts of surfactant-associated proteins and phospholipids become prominent in the distal epithelium. Differentiation of squamous type I cells from type II cells begins. A dramatic expansion of the pulmonary capillary bed (vascular canals) in the lung parenchyma gives this stage its name (see Fig. 2-1D ). These vessels surround the developing acini and come in direct contact with the epithelium, giving rise to the primordial air-blood barrier.
During the saccular stage, which persists from week 24 until term, the terminal acinar tubules in the lung periphery continue to branch and air space size increases. Alveolar type II cells undergo significant maturation, as evidenced by increased synthesis of SP-A, SP-B, SP-C, and SP-D and of surfactant phospholipids. Glycogen stores, which serve as a substrate for phospholipid synthesis, decrease, while the number of lamellar bodies increases. Squamous type I cells continue to differentiate and constitute an increased proportion of the distal lung surface, thereby increasing the effective area for gas exchange (see Fig. 2-1E ). Septal walls consist of a central connective tissue core with a capillary network on each side. Subsequent fusion of the basal laminae of the distal epithelium and endothelium brings capillaries into close association with type I cells, which decreases the diffusion distance between air spaces and capillaries to allow more efficient gas exchange (see Fig. 2-1E ).
The transition from the canalicular to saccular stage of lung development marks the threshold of viability for preterm infants who have access to neonatal intensive care support. Before 22 weeks’ gestation there is insufficient surface area in the distal pulmonary tree to support safe, reliable oxygenation and ventilation, even when surfactant replacement therapy and sophisticated mechanical ventilation techniques are available. Survival at 23 weeks’ gestation ranges from 15% to 30%. Mortality decreases with each additional week of gestation; by 25 weeks, survival exceeds 60%, although significant morbidity in the form of bronchopulmonary dysplasia (BPD) and neurodevelopmental compromise persists.
As the threshold of viability is crossed, respiratory distress syndrome (RDS) becomes the primary source of morbidity and mortality for the preterm infant. RDS is a consequence of deficient surfactant production, leading to terminal airway atelectasis and epithelial injury. The subsequent capillary leak produces the hyaline membranes that are classically associated with this disease. Surfactant replacement therapy has dramatically improved RDS survival rates and reduced morbidity. Term infants affected by RDS often have comorbid conditions such as maternal diabetes, which delay maturation of the surfactant production system.
Genetic mutations leading to SP-B deficiency result in a clinical presentation indistinguishable from the early stages of RDS. Affected infants, however, are typically full term and have only a transient response to surfactant replacement therapy, which leads to early neonatal death or the development of severe neonatal chronic lung disease. The only definitive treatment is lung transplantation. Lethal respiratory failure in a mouse model of SP-B deficiency can be reversed with targeted expression of an SPB transgene, demonstrating the potential for gene therapy. Mutations of SPC produce a spectrum of pulmonary disorders during infancy, including interstitial pulmonary fibrosis. As with SP-B deficiency, surfactant replacement therapy has little or no benefit, with lung transplantation as the only documented potential cure. Other genetic defects of the surfactant production system also lead to fatal surfactant deficiency in the neonate. Infants deficient in ABCA3, which transports surfactant phospholipids to lamellar bodies, have normal SP-B expression but develop unexplained lethal respiratory failure and death within 1 month of birth.
The final stage of lung development is the alveolar stage, which lasts from week 36 of gestation through the first 18 months of postnatal life. As the name implies, true alveoli are generated from terminal saccules during this stage. Interstitial tissue in primary septa is reduced, while secondary septa markedly lengthen and thin (see Fig. 2-1F ). Concomitant with these changes is the fusion of the double septal capillary network into one (see Fig. 2-1F ). This remodeling requires an initial burst of interstitial fibroblast proliferation, which subsequently slows down, and the cells synthesize increased amounts of collagen and elastin. Septation results in a marked increase in the number of alveoli from approximately 30 million at term to 300 million in the adult. Increased numbers of type II and type I cells accompany alveolar expansion, with type I cells now covering 95% of the alveolar surface area.
BPD, which is typically restricted to infants born before 32 weeks’ gestation, represents a particularly challenging complication. The condition is only associated with preterm birth and is defined by a characteristic appearance on chest radiograph and persistent requirement for supplemental oxygen beyond 36 weeks after conception. In the era of surfactant replacement therapy, BPD is distinguished by alveolar simplification due to the apparent arrest of the alveolarization during the third trimester. Compromised oxygenation and ventilation may worsen as infant somatic growth progresses and attendant metabolic demands outstrip pulmonary function. Respiratory morbidity is not restricted to infants born before 32 weeks’ gestation. Late-preterm infants born between 32 and 37 weeks’ gestation are more likely than term infants to require respiratory support, including positive-pressure ventilation, after birth. Given the dramatic increase in alveolar number during the late third trimester, it follows that late-preterm infants may have a smaller margin of safety when making the transition to extrauterine life.
Tissue Interactions and Lung Development
A basic tenet of lung development is that it requires inductive interactions between the endodermal epithelium and the mesodermal mesenchyme. Reciprocal inductive interactions involve one cell type signaling to another cell type and then responding to signals sent back; both cell types are thus signaling and responding to each other. This is particularly evident in the embryonic and pseudoglandular stages, where it has been shown conclusively that lung epithelium must be associated with lung mesenchyme in order to survive and branch. The factors that drive branching morphogenesis are diffusible, because embryonic lung epithelium branches when separated from lung mesenchyme by a filter that prevents direct cell-cell contact but allows diffusion of soluble factors. Importantly, these experiments also showed that survival of lung mesenchyme is dependent on the presence of lung epithelium, underscoring that induction is reciprocal. The fate of the entire respiratory endoderm, from the trachea to the bud tips, however, is not fully committed during the embryonic stage. Reciprocal recombination experiments have shown that distal lung mesoderm can reprogram tracheal endoderm to branch and differentiate like lung, and that tracheal mesoderm can reprogram lung endoderm to differentiate like trachea.
Bronchopulmonary sequestration may represent an intriguing manifestation of aberrant pulmonary endoderm-mesoderm interaction. These masses of abnormal lung tissue, which may be contained within the lung or in an extrapulmonary location within the abdomen, may be in direct communication with gastrointestinal tract structures, suggesting ectopic induction of embryonic foregut. The histopathologic appearance of these lesions includes typical cellular components of pulmonary parenchyma along with inflammatory and fibrotic components ( Fig. 2-2C ).

Normal lung function requires the precise alignment of the distal epithelium and the vasculature to meet the respiratory requirements of the developing organism. Certain lethal congenital malformations of the lung, such as alveolar capillary dysplasia with misalignment of pulmonary veins (ACD/MPV), are due to a perturbation in the relationship between vascular and airway development. ACD/MPV is characterized by a paucity of alveolar capillaries, thickened pulmonary mesenchyme, and misalignment of the pulmonary veins, which reflect the reciprocal relationship required for airway and vascular development (see Fig. 2-2B ). ACD/MPV is associated with mutations in the transcription factor FOXF1, which is expressed in the lung mesenchyme. The alveolar simplification of BPD is also accompanied by a relative paucity of alveolar capillaries that is reminiscent of ACD/MPV. Evidence that vascular endothelial growth factor A (VEGF-A), which is produced by lung epithelial cells, can reverse the alveolar defect further reinforces the concept of interdependent development of vascular and airway structures.
Molecular Regulation of Lung Development
Elucidation of the factors that regulate lung growth and development has been the focus of an intense research effort. This stems not only from a desire to understand the basis of pulmonary pathologic conditions present at birth, but also from the possibility that understanding how the lung develops will provide insight into how the lung repairs itself following injury or disease. Given the morphogenetic precision required to generate a lung that can function effectively in gas exchange, coupled with the fact that the lung contains over 40 differentiated cell types, it is not surprising that the molecular regulation of lung development is proving to be very complex. Identifying the factors involved provides only part of the story. When, where, how much of, and for how long these factors are expressed must also be considered. The fact that there is crosstalk between some of the identified pathways significantly increases the level of complexity.
Diffusible Mediators of Lung Development
Fibroblast Growth Factors and Fibroblast Growth Factor Receptors
In both humans and mice, the fibroblast growth factor (FGF) family comprises 22 structurally related molecules ; among these, FGF1, 2, 7, 9, 10, and 18 have been localized to the developing lung. FGFs bind and signal through high-affinity, ligand-dependent transmembrane receptors ( fibroblast growth factor receptors [FGFRs]) that contain an intracellular tyrosine kinase domain. There are four FGFRs, all of which are expressed in the lung. Alternative messenger RNA splicing results in two isoforms each for FGFR1, FGFR2, and FGFR3 that have distinct ligand specificities. FGFR activation is modulated by heparin or heparan sulfate.
FGF1 and FGF2 are not critical for lung development, because the single deletion of either gene or the double ablation of both has no effect on lung development. FGF10 is an ideal candidate for mediating tissue interactions in the lung, because it is expressed in the mesenchyme, whereas its primary receptor, FGFR2b, is expressed by epithelial cells. Ablation of either FGF10 or FGFR2b results in complete pulmonary agenesis caudal to the trachea. The basis for this phenotype comes from the ability of FGF10 to induce lung epithelial budding by chemoattraction ; in the absence of FGF10, primary buds cannot form. FGF10 affects the expression of many target genes in early lung epithelium, including other important signaling molecules such as bone morphogenetic protein 4 (BMP4) and Notch family members.
Like FGF10, FGF9 acts as a mediator of reciprocal tissue interactions, because it is expressed in the epithelium and mesothelium, whereas its receptor (FGFR2c) is found in the mesenchyme. FGF9 controls lung mesenchyme size by regulating cell proliferation. The observation that the amount of available mesenchyme appears to control lung branching is consistent with the finding that the lungs of Fgf9 -null mice are severely hypoplastic, with decreased amounts of mesenchyme and reduced Fgf10 expression. The observation that Fgf18 -null mice exhibit reduced alveolar size resulting from reduced cell proliferation during the saccular stage suggests a role for FGF18 in late lung development. FGF7 has been shown to stimulate lung epithelial cell proliferation, as well as surfactant protein gene expression and surfactant phospholipid synthesis in type II cells. Transgenic overexpression of Fgf7 in the developing mouse lung epithelium results in lesions resembling congenital cystic adenomatoid malformations ; examination of human congenital cystic adenomatoid malformations, however, shows that FGF7 expression is actually decreased and FGF10 expression is unchanged. Although intraperitoneal injection of neutralizing antibodies against Fgf7 inhibits postnatal lung growth and alveolus formation, mice with a targeted deletion of Fgf7 have no apparent lung phenotype. Other FGFs are also required during alveologenesis, because mice with deletions of both Fgfr3 and Fgfr4 fail to form normal alveoli.
The Sprouty (SPRY) proteins, which antagonize FGFR signaling, modulate the effects of FGFs in the developing lung. Although single deletion of either Spry2 or Spry4 has no effect on lung development, mice null for both genes have defects in multiple organs, including the lung.
Retinoic Acid
Retinoic acid (RA), the active derivative of vitamin A, is essential for the normal development of many tissues, including the lung. Maternal vitamin A deficiency results in severe respiratory phenotypes in offspring, including tracheoesophageal fistula, lung hypoplasia, and lung agenesis. RA signals through RAR and RXR nuclear receptors, both of which have α, β, and γ isoforms, and these are expressed in the lung from the outset of development. Mice with double deletions of Rara/Rarb or Rara/Rxrb show the same lung abnormalities as those seen in vitamin A–deficient embryos. The mechanism by which RA controls lung morphogenesis is not fully resolved. Data from cultured early embryonic foreguts suggest that RA allows activation of Wingless (Wnt) signaling by inhibiting Dickkopf1 (DKK1); this affects FGF10 expression in the mesoderm, as well as maintenance of lung progenitor cell fate. RA further affects lung bud induction by inhibiting transforming growth factor-β (TGF-β) activity in the prospective lung field, which in turn allows expression of FGF10.
RA also enhances perinatal alveolus formation in rodents, which has led to its clinical use for the prevention of BPD. The effect is modest but significant; about 15 infants must be treated to prevent one case of BPD. The mechanism is not understood in detail but is likely related to maintenance of alveolarization after preterm delivery, reducing the potential for alveolar simplification.
Sonic Hedgehog
The hedgehog signaling pathway plays an important role in the development of multiple organs. Sonic hedgehog (SHH) is highly expressed in the developing lung epithelium, and its primary receptor, patched 1 (PTCH1), is found in mesenchymal cells, suggesting that SHH is part of an epithelial-mesenchymal inductive loop. Shh is initially expressed throughout the epithelium but becomes restricted to subsets of cells from day E16.5 onward. Shh -null mice form lungs, indicating that Shh is not required for lung specification and bud induction; however, these lungs are severely hypoplastic, suggesting that Shh is involved in regulating branching morphogenesis. Shh deletion profoundly affects lung growth and patterning, but the specification of epithelial cell types appears to be unaffected. Because Shh serves as a survival factor for lung mesenchymal cells, the lung hypoplasia seen in Shh- null embryos may be due to a decrease in mesenchymal mass. Shh is also a negative regulator of Fgf10, and Shh- null embryos exhibit expanded Fgf10 expression. A clinical syndrome with a respiratory phenotype that is consistent with disruption of SHH signaling is Smith-Lemli-Opitz. Smith-Lemli-Opitz syndrome is phenocopied by mutations in Δ-7-dehydrocholesterol reductase ( DHCR7 ), which is involved in cholesterol synthesis ; cholesterol modification of SHH is required for effective signaling.
SHH levels are modulated by its binding to PTCH1. In the absence of ligand, PTCH1 represses Smoothened (SMO) and prevents activation of the hedgehog signaling pathway. SHH also up-regulates PTCH1 expression, and any PTCH1 in excess of that involved in controlling signaling binds SHH and sequesters it, creating a negative feedback loop that restricts its spread. Another molecule regulating SHH levels is hedgehog interacting protein (HHIP), a membrane-bound protein that binds all mammalian hedgehog proteins and, like PTCH1, is up-regulated in response to SHH. Targeted deletion of HHIP results in lung hypoplasia that may be due to a loss of FGF10 expression at the prospective sites of bud formation as a result of increased SHH signaling.
Transforming Growth Factor – β Superfamily
The TGF-β superfamily comprises activins, inhibins, the BMPs, müllerian inhibiting substance, and TGF-β1, 2, and 3. TGF-β1 treatment of cultured embryonic lung explants or misexpression of TGF-β1 targeted to the lung in vivo severely inhibits branching morphogenesis. This is likely due to the ability of TGF-β1 to inhibit FGF10 expression. TGF-β1 signals through a heteromeric complex of type I (TGF-βrI) and type II (TGF-βrII) receptors and exerts its effects on downstream target genes via the Smad family of proteins. Inhibition of TGF-βrII in cultured embryonic lungs increases lung branching, as does attenuation of SMAD2/3, underscoring the inhibitory nature of TGF-β1 on lung morphogenesis. Tgfb1 -null mice show no apparent lung phenotype, although it should be noted that 50% of these mice die on E10.5, just after the onset of lung development. Most Tgfb2 -null mice die shortly before or during birth with a wide range of developmental defects. The lungs of neonates have dilated conducting airways and collapsed terminal and respiratory bronchioles. Deletion of Tgfb3 results in retarded development and differentiation of the lung epithelium, mesenchyme, and vasculature. The fact that TGF-β3 appears to promote morphogenesis contrasts with the inhibitory function of TGF-β1, suggesting that these ligands affect distinct aspects of lung development.
Of the four BMPs expressed in the developing lung (BMP3, 4, 5, and 7), BMP4 has been the focus of the most studies. Bmp4 is expressed in the ventral foregut mesenchyme before lung bud induction and then is expressed in the distal epithelium and proximal mesenchyme after the lung has formed. In the mouse, epithelial expression declines in the distal epithelium before birth but begins in the capillary endothelium. Bmp4 expression is up-regulated by Fgfs in the epithelium and by Shh in the mesenchyme. Specific deletion of Bmp4 or BMP receptor 1a ( Bmpr1a ) from the distal lung epithelium results in reduced proliferation, increased apoptosis, and cystic morphogenesis. Early endodermal deletion of both Bmpr1a and Bmpr1b results in reduced ventral Nkx2.1 expression, which is replaced by expanded expression of dorsal Sox2. These data support a model in which BMP4 promotes the proliferation and survival of undifferentiated lung progenitor cells.
Wnts and β-Catenin
Members of the Wnt family of secreted glycoproteins are critically involved in cell fate determination, proliferation, survival, and motility in organogenesis. Wnt ligands bind their receptors to activate a pathway that ultimately stabilizes β-catenin, which then interacts with nuclear T-cell factor/lymphoid enhancer factor (TCF-LEF) transcription factors to modulate transcription of downstream target genes. Wnts1, 2, 2b, 5a, 7b, and 11 are expressed in the lung. Their secretion is mediated by the transmembrane protein Wntless (WLS); deletion of WLS from the lung endoderm disrupts branching morphogenesis and pulmonary endothelial differentiation. Canonical Wnt signaling plays a critical role in lung development, because endodermal deletion of β-catenin abrogates specification of lung progenitors and leads to complete lung agenesis. The ligands responsible for lung progenitor specification are likely Wnt2/2b, because their dual deletion phenocopies exactly the endodermal loss of β-catenin. Proximal-distal airway patterning and epithelial cell differentiation are disrupted when Wnt signaling is inhibited after specification of lung progenitors, either by targeted epithelial deletion of β-catenin or by misexpression of the Wnt antagonist Dkk1. In addition to its role in specifying lung endoderm, Wnt2 also activates a signaling network necessary for smooth muscle differentiation. Inactivation of Wnt5a results in a foreshortened trachea, distended distal airways, and retarded lung maturation. Mice null for Wnt7b die at birth from respiratory failure. Early proliferation is reduced in both epithelial and mesenchymal tissue compartments, leading to lung hypoplasia, although cell fate specification and overall tissue architecture are unchanged. Constitutive activation of Wnt signaling in the developing lung epithelium with hyperactive β-catenin results in lungs that lack fully differentiated cell types and instead contain multiple intestinal and nonlung secretory cell types. Taken together, these observations indicate that the temporospatial regulation of Wnt signaling must be tightly regulated to ensure normal lung morphogenesis and differentiation.
Platelet-Derived Growth Factor
The platelet-derived growth factor (PDGF) family consists of five different disulphide-linked dimers built up of four different polypeptide chains encoded by four different genes. PDGF-A, which homodimerizes with itself or heterodimerizes with PDGF-B, plays an important role in lung development. PDGF-A is expressed in distal lung epithelium, whereas its receptor, PDGFRA, is expressed in nearby mesenchymal cells, indicative of a paracrine signaling loop between the epithelium and mesenchyme. Deletion of PDGF-A results in arrested alveolus formation and postnatal death. The lungs lack the differentiated alveolar myofibroblasts that produce elastin, which is critical for alveolus formation.
Vascular Endothelial Growth Factor
VEGF-A, C, and D are all found in the lung. The temporal and spatial expression of VEGF-A during lung development implies a central role in the maturation and organization of the pulmonary vascular network. VEGF-A is expressed in epithelial and mesenchymal compartments during the embryonic and pseudoglandular stages, becoming more restricted to the epithelium as development progresses into the canalicular stage. VEGF-A exists as three isoforms (120, 164, and 188) that have distinct functions in vascular development. Genetic studies in mice demonstrate the importance of local tissue concentrations of Vegf-a to effect appropriate vascular development and distal airway structures. Increased expression of Vegf164 in distal epithelium disrupts assembly of the vascular plexus and arrests airway branching without affecting endothelial cell proliferation or survival, indicating that crosstalk between the developing epithelium and vasculature is required for normal morphogenesis. Vascular ablation in the early lung causes significant alterations in stereotypic branching of the epithelium. VEGF-A expression is controlled by multiple mediators, such as FGFs and SHH.
Glucocorticoids
Glucocorticoids exert potent effects on a variety of different tissues, with a common theme that they induce the precocious appearance of normal developmental events. The effects of glucocorticoids on lung function have been a topic of intense interest since the observation that dexamethasone accelerates lung maturation in premature lambs. Glucocorticoid receptors are present on the developing pulmonary epithelium as airway branching progresses during the pseudoglandular stage of lung development. Epithelial expression persists through the saccular and alveolar stages, accompanied by the onset of expression within the mesenchymal compartment. Exogenous glucocorticoids stimulate morphologic maturation and many aspects of surfactant phospholipid biosynthesis. Targeted disruption of the glucocorticoid receptor in mice leads to respiratory distress and early neonatal death ; the lungs of these animals are atelectatic with blunted alveolarization. Although the number of type II cells is increased by 30%, the relative expression of Sp-a and Sp-c is decreased by 50%. The number of type I cells is decreased by 50%, as are the type I cell markers T1α and aquaporin-5, suggesting that glucocorticoids facilitate the differentiation of type II cells into type I cells. Somewhat paradoxically, however, mice null for corticotropin-releasing hormone show deficits in septal thinning, air space formation, and content of Sp-a and Sp-b but have no deficit in surfactant phospholipid biosynthesis.
Given the broad distribution of pulmonary glucocorticoid receptors in the developing lung, it is not surprising that the therapeutic effects of glucocorticoid treatment are complex. Clinical experience suggests that glucocorticoids have contrasting biologic effects depending upon whether treatment is directed toward the fetal lung or the preterm neonatal lung. Women in preterm labor are routinely treated with glucocorticoids to reduce the incidence and severity of neonatal RDS. Antenatal steroid treatment accelerates fetal lung maturation by inducing mesenchymal thinning and enhancing pulmonary function, presumably through stimulation of surfactant production. Morphometric studies in sheep suggest that antenatal steroid treatment may also induce some blunting of alveolarization. Glucocorticoid treatment has also been employed to treat preterm infants experiencing severe BPD. Although early studies and anecdotal reports suggested that steroid treatment could reverse the fibrosis and scarring associated with BPD and significantly improve pulmonary mechanics, subsequent studies demonstrated no clear improvement in long-term pulmonary outcome and increased risk for neurodevelopmental impairment.
Transcriptional Regulation of Lung Development
The diffusible molecules mediating tissue interactions in the developing lung initiate signaling cascades that lead to changes in gene expression. The diversity of cell types found in the lung, which all differentiate under tight spatial and temporal control, makes regulation of gene expression by transcription factors in the developing lung highly complex. Although no lung-specific transcription factors have yet been found, research over the last decade has identified several transcription factors in addition to those described earlier that are crucial to normal lung development.
NKX2-1
NKX2-1 (also known as “thyroid transcription factor 1” [TTF1]) is found in the presumptive respiratory region of the foregut endodermal epithelium before lung bud induction. NKX2-1 is expressed in the forebrain, thyroid, and lung, where it interacts with multiple partners to influence several key aspects of development. Mice null for Nkx2-1 develop tracheoesophageal fistulas, with main-stem bronchi connecting to hypoplastic, cystic lungs. Whereas differentiation of the most proximal epithelium is somewhat preserved in Nkx2-1 –null lungs, markers of distal epithelial differentiation, including the surfactant proteins, are completely lacking. Haploinsufficiency for the NKX2-1 gene in humans leads to brain-lung-thyroid syndrome, which is characterized by benign hereditary chorea, respiratory disease, and congenital hypothyroidism. The respiratory phenotypes include RDS at birth, as well as recurrent pulmonary infections and interstitial lung disease later in childhood. The control of NKX2-1 expression in lung development is not fully understood.
GLI Genes
Three GLI genes (1, 2, and 3) code for zinc finger transcription factors that are the principal effectors of hedgehog signaling. All three Gli genes are expressed in distinct but overlapping domains in lung mesenchyme, with expression being highest in the distal tips. The analysis of compound mutant mice has demonstrated the complexity of how Gli genes affect lung development. Embryos expressing different combinations of Gli genes show a range of lung defects, the most striking of which is the absence of lungs, trachea, and esophagus in Gli2 -/- , Gli3 -/- compound mutants. The presence of a single Gli3 allele ( Gli2 -/- , Gli3 +/- ) is sufficient to allow formation of hypoplastic lungs in which the left and right lungs do not separate, and the embryos have tracheoesophageal fistulas. The phenotype seen in Gli2/Gli3 double-null embryos is more severe than that seen in Shh -null animals; this suggests that the GLI genes may lie downstream in signaling pathways other than SHH, or that the other hedgehog proteins (Indian and desert) may be active in the lung. Mutations in the human GLI3 gene cause Pallister-Hall and Greig syndromes, which affect development of several organ systems, including the lung.
FOX Family
The FOX family of transcription factors contains more than 50 members, all of which share a winged-helix DNA binding domain. FOXA1 and FOXA2 are closely related proteins found in the foregut endoderm and its derivatives. Their spatial and temporal expression patterns are similar in the lung. Mice lacking Foxa2 do not form endoderm and hence cannot form lungs ; targeted deletion of Foxa2 in lung epithelial cells, however, demonstrates that it is required for alveolarization and epithelial cell differentiation. Deletion of Foxa1 in mice delays some aspects of sacculation and alveolarization prenatally and perinatally, but these differences normalize by 2 weeks of age, suggesting compensation by Foxa2 . Deletion of both genes inhibits cell proliferation, branching morphogenesis, and epithelial cell differentiation, indicating that FOXA1/2 play a central role in lung development.
Foxa1 is expressed in lung mesenchyme and controls genes involved in epithelial-mesenchymal interactions, because a haploinsufficiency results in defective branching, lobation, and epithelial differentiation in the mouse lung. In humans, FOXF1 mutations are associated with ACD/MPV. Foxj1 controls expression of left-right dynein, which is required for correct anchoring of basal bodies; deletion of Foxj1 causes situs inversus, the loss of motile cilia in airway epithelial cells, sinusitis, and bronchiectasis. Although these features are associated with Kartagener syndrome in humans, no mutations in the FOXJ1 gene have been directly linked to this disorder. FOXP1 and FOXP2, which are known transcriptional repressors, are expressed in the lung epithelium; both genes are expressed distally, but only FOXP1 is expressed proximally. Foxp2 -/- mice show impaired alveolarization, an effect exacerbated in compound mutant Foxp2 -/- , Foxp1 +/ – mice, which have hypoplastic lungs and die at birth. Foxp1 acts cooperatively with Foxp4 to restrict goblet cell specification, thereby regulating the balance of cell types in the airway epithelium.
GATA6
GATA6, a zinc finger transcription factor that is required for visceral endoderm differentiation, is the only GATA family member expressed in the distal epithelium of the developing lung. Mice bearing a dominant-negative Gata6/engrailed fusion protein under control of the Sftpc promoter show reduced numbers of proximal airway tubules. Lung epithelial cell differentiation is also affected, with these mice completely lacking detectable alveolar type I cells. Loss of Gata6 in the lung epithelium causes a loss of differentiation and the precocious appearance of bronchioalveolar stem cells that is the result of increased Wnt signaling. GATA6 regulates expression of WNT7B and also interacts with NKX2-1 to control expression of SP-A, B, and C.
SOX Family
Members of the SOX family of transcription factors function as key regulators of cell fate and differentiation. Of the 20 known SOX proteins, SOX2, 4, 9, 11, and 17 are found in the developing lung. Sox2 is highly expressed in nonbranching epithelium but repressed by Fgf10 in epithelial cells that are actively invading the surrounding mesenchyme, suggesting that silencing of Sox2 is required for the epithelium to branch. The repression of Sox2 by Fgf10 may be mediated by BMP signaling. Overexpression of Sox2 in lung epithelial cells inhibits lung branching by forcing the cells to commit prematurely to a differentiation program, thereby rendering the cells incompetent to respond to branching signals. Sox11 is also expressed throughout the developing lung epithelium, and mice null for Sox11 have significant lung hypoplasia. Sox17 expression in the lung is dynamic, being first detected in the mesenchyme during the embryonic stage, then in the conducting airway epithelium during the canalicular stage. Because its misexpression in the distal epithelium disrupts branching and causes the ectopic expression of proximal airway markers, Sox17 is thought to play a key role in specifying differentiation of airway epithelial cells.
Posttranscriptional Gene Regulation in Lung Development
Micro-RNAs (miRNAs) are small noncoding RNA molecules that modulate physiologic and pathologic processes by inhibiting gene expression through RNA translation repression or messenger RNA degradation. Functionally mature miRNAs are generated by a series of ribonuclease III cleavage steps. Key enzymes in miRNA biogenesis include DROSHA, which cleaves primary miRNAs into precursor miRNAs in the nucleus, and DICER1, which cleaves precursor miRNAs to the mature form in the cytoplasm. Mature miRNAs are incorporated into the large multiprotein RNA-induced silencing complex, which represses RNA translation or induces messenger RNA degradation. miRNAs regulate key biologic processes important in lung development, including cellular proliferation, apoptosis, and differentiation. miRNA profiling reveals that the lung has a specific miRNA expression profile that is conserved across species (including mouse and human) and regulated specific to developmental stage, sex, and cell type. miRNAs have a critical role in controlling organogenesis. Loss- and gain-of-function studies, as well as differing expression profiles between patients or animal models with lung disease and normal controls, implicate miRNAs in the pathogenesis of many lung diseases, including chronic obstructive pulmonary disease, lung cancer, pulmonary inflammatory disease, idiopathic pulmonary fibrosis, asthma, and cystic fibrosis (for reviews see references ). Additionally, studies in model systems have identified a critical role for miRNA-mediated regulation in lung development. Inactivation of Dicer1, a key enzyme in miRNA biogenesis, targeted to the developing lung epithelium results in neonatal death because of arrested airway-branching morphogenesis, increased cell death, and altered expression of critical epithelial-mesenchymal signaling molecules. Specific miRNAs that have been identified to influence lung development include the miR302/367 cluster that directs lung endoderm development by coordinating proliferation, differentiation, and apical-basal polarity of lung progenitor cells, the miR17-92 cluster that is required for lung growth as well as for promoting proliferation and inhibiting differentiation of lung epithelial progenitor cells, miR127, which regulates terminal bud size and number, and miR221 and miR130a, which have opposing effects on airway and vascular morphogenesis. The recent discovery of heterozygous germline loss-of-function DICER1 mutations in familial pleuropulmonary blastoma (PPB), a rare pediatric lung tumor that often arises during lung development, provides evidence that the DICER1 /miRNA pathway controls human lung development and suppresses tumorigenesis. The majority of carriers with DICER1 mutations are phenotypically normal, suggesting that loss of one DICER1 allele is compatible with normal development and insufficient for tumor formation. PPB is composed of both epithelial and mesenchymal cells. In a subset of patients, overgrowth of the mesenchymal cells results in a sarcoma that is associated with a poorer prognosis. Interestingly, the protein DICER1was found to be lost in the epithelial tumor component but retained in the mesenchymal cells by immunohistochemistry, suggesting that loss of DICER1 specifically in the lung epithelium promotes PPB formation. Consistent with this notion, Dicer1 gene ablation targeted to the developing murine pulmonary epithelium results in a PPB-like phenotype ( Fig. 2-3 ). Because PPB often arises in the setting of an inherited tumor predisposition syndrome characterized by increased incidence of other neoplasms, including cystic nephroma, ovarian sex cord–stromal tumor, embryonal rhabdomyosarcoma, and multinodular goiter, the DICER1/miRNA pathway functions that control lung development are probably also operative in other organs.
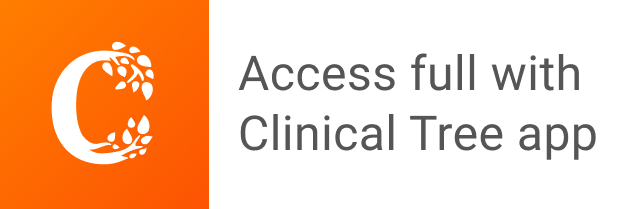