Introduction
- Kazuaki Negishi, MD, PhD
- Thomas H. Marwick, MD, PhD, MPH
The assessment of global left ventricular (LV) systolic function is a cornerstone of risk evaluation and management in most cardiac diseases. The simplest and most widely used parameter for this purpose has been LV ejection fraction (EF) and regional wall motion analysis, but over the last decade, new parameters such as strain imaging have become available.
Indications for systolic function evaluation
Echocardiography is appropriately indicated when cardiac-related symptoms or conditions are suspected, most commonly in the setting of suspected heart failure (HF). Despite the value of LV dysfunction as an important component of risk evaluation for decision making, the methodologies and cutoffs used in studies that have defined these EF criteria for the guidance of management are highly variable, with few studies using core laboratories ( Table 27.1 ). The EF criteria for recommending treatment with implantable defibrillators and cardiac resynchronization therapy, or intervention in regurgitant valve lesions have not been gathered with a high degree of accuracy, and it seems irrational to use them as exact thresholds.
Study | Intervention | Number | Technique | Core Lab | Entry Criteria |
---|---|---|---|---|---|
SOLVD, 1991 | Enalapril | 2569 | Echo, RNV, LVgram | Yes | EF ≤ 35% |
Hydralazine-nitrate, 1991 | Enalapril vs. hydralazine-nitrate | 804 | Echo, RNV | Yes | EF < 45%, LVEDD > 27 mm/m 2 BSA |
CIBIS, 1994 | Bisoprolol | 641 | RNV, LVgram | No | EF < 40% |
US Carvedilol, 1996 | Carvedilol | 1094 | Echo | Yes | EF ≤ 35% |
MERIT-HF, 1999, 2000 | Metoprolol XL | 3991 | Unspecified | No | EF ≤ 40% |
CIBIS II, 1999 | Bisoprolol | 2647 | Echo, RNV, LVgram | No | EF ≤ 35% |
Capricorn, 2001 | Carvedilol | 1959 | Echo, RNV, LVgram | No | EF ≤ 40%, WMSI ≤ 1.3 |
Carvedilol, 2001 | Carvedilol | 2289 | Unspecified | No | EF < 25% |
BEST, 2001 | Bucindolol | 2708 | RNV | No | EF < 35% |
MIRACLE-ICD, 2003 | CRT/ICD | 369 | Echo | Yes | EF ≤ 35% |
COMET, 2003 | Carvedilol vs. metoprolol | 1511 | Echo, RNV | No | EF < 35% |
CHARM, 2003 | Candesartan | 2548 | Unspecified | No | EF ≤ 40% |
SCD-HeFT, 2005 | ICD | 3521 | Unspecified | No | EF < 35% |
CARE-HF, 2005 | CRT | 813 | Echo | Yes | EF ≤ 35%, LVEDD > 30 mm/m height |
In asymptomatic subjects at risk for heart failure, the use of echocardiography to detect reduced EF or subtle structural heart disease reclassifies the patient from stage A to stage B of heart failure, with resulting management implications. These patients with a subclinical phase to their cardiomyopathy include those treated with cardiotoxic chemotherapy, and those with gene-positive cardiomyopathies, amyloidosis, or other infiltrative conditions. The detection of subclinical disease not only includes global but also regional dysfunction (in ischemic heart disease, sarcoidosis, and myocarditis).
Finally, although the temporal analysis of LV contraction carries prognostic information, the clinical application of this approach remains uncertain. There are very strong prognostic reasons to undertake cardiac resynchronization therapy in patients with heart failure symptoms, systolic dysfunction, and left bundle branch block, regardless of the measurement of mechanical synchrony, and there are reasonable grounds to doubt the reliability of some of the literature on prediction of cardiac resynchronization therapy response. Paradoxically, measurement of synchrony may come into clinical use in the selection of patients for implantable defibrillators rather than cardiac resynchronization, because measurements of dispersion of mechanical activation may be of value in understanding the risk of arrhythmia.
Limitations of ejection fraction
In spite of the breadth of its use, EF has a number of important limitations ( Table 27.2 ). Some of these, such as the use of a variety of geometric assumptions and the error introduced by tangential tomographic planes, generally pose a greater problem to the evaluation of LV volumes than EF because the errors seem to cancel out in the evaluation of EF. Ejection fraction is load dependent, meaning that it cannot be interpreted as a reflection of contractility in the absence of knowledge about afterload and preload. Although properties of an ideal index of contractility might include sensitivity to changes in inotropy; independence of load, heart size, and mass; ease and safety of application; and proof in the clinical setting, no perfect index currently exists. Perhaps the best way to integrate the role of loading in the evaluation of LV function is to ensure that the measurement of blood pressure (as an analogue of central pressure) and to a lesser extent, inferior vena cava characteristics, should be included in imaging studies that could be used clinically to estimate contractility.
Problem | Circumstances of Inaccuracy | Potential Solution |
---|---|---|
Geometry dependence | LBBB, extensive wall motion abnormality, off-axis imaging | 3D imaging, geometry-independent techniques |
Load dependence | Extremes of afterload, mitral regurgitation | Pressure volume loops, preejection markers |
High and low HR | Heart block, tachycardias (especially atrial fibrillation) | None |
Marker of endocardial shortening | LV hypertrophy | Midmyocardial shortening |
Insensitivity to minor change | Prognostic value close to EF 50% | Non-EF techniques for assessing subclinical dysfunction |
Expertise | Wide use of EF | Quantitation |
Ejection fraction is also influenced by heart rate. The increased stroke volume associated with bradycardia may lead to overestimation of the true EF; and conversely, in tachycardia, the reduced stroke volume may lead to underestimation of the actual function. There is no technique that corrects for this, so heart rate must also be included in the interpretation of EF data.
Ejection fraction is a function of LV size. In LV hypertrophy, EF may not correspond well to the real interest of the clinician, which is function in the midmyocardium. Furthermore, although EF is a good marker of gross LV dysfunction, it may be insufficiently sensitive to identify mild degrees of systolic dysfunction, perhaps evidenced by the inability to identify a gradation of risk in patients with EF greater than 45%. In contrast, the use of global longitudinal strain offers the greatest increment in predictive power in patients with EF greater than 35%, and without wall motion abnormalities. Finally, the wide use of EF suggests that not all assessments are performed by practitioners with the same level of expertise, indicating that more formal quality control, automation, and quantitation may be desirable.
Choice for assessment tool of LV systolic function in multimodality ERA
LV systolic function is currently assessed with various modalities. The right tool can be selected by understanding the fundamental characteristics of each modality ( Table 27.3 ). These include spatial, temporal, and contrast resolution; three-dimensional (3D) acquisition and display; repeatability; and intraobserver and interobserver variation.
Technique | Application | |
---|---|---|
High spatial resolution | CMR | LV hypertrophy, infiltration |
High temporal resolution | Tissue Doppler, strain | LV synchrony |
High contrast resolution | CMR, contrast echo | LV volumes |
High repeatability | CMR, 3D echo | Sequential follow-up |
Sensitivity to minor change | Strain | Subclinical cardiomyopathy |
Echocardiography, cardiac magnetic resonance (CMR), and computed tomography (CT) have a spatial resolution of a millimeter or a few millimeters. In contrast, nuclear cardiology techniques have a lower spatial resolution of up to 1.0 cm. Techniques with lower spatial resolution are inherently less suited to the generation of exact measurements of regional wall motion disturbances, wall thickness, and dimension.
The highest temporal resolution is achieved with echocardiographic techniques. Generally, this component of imaging is considered important when assessing the rate of contraction or the presence and degree of LV dyssynchrony. This underlies the assessment of LV synchrony with techniques of low temporal resolution, such as 3D echocardiography and single photon emission computed tomography. Another aspect of temporal resolution, which is often neglected, is the importance of measuring and averaging a large number of cardiac cycles in patients with stable cardiac rhythm. Failure to do so is one of the weaknesses of echocardiography, which then exposes measurements to the risk of sampling error based on individual cardiac cycles.
Contrast resolution is the ability to accurately distinguish the LV wall from the blood pool. The best contrast resolution is obtained with magnetic resonance imaging. Contrast techniques such as contrast echocardiography or ventriculography improve the contrast resolution of the underlying technique. These considerations are vital in the accurate measurement of LV dimensions and volumes. From the standpoint of contrast resolution, as well as tissue characterization, magnetic resonance imaging is the optimal tool.
Three-dimensional imaging is available with echocardiography, CMR, and CT. The main attraction of 3D imaging is its intrinsic ability to avoid making geometric assumptions when calculating LV volumes, and to avoid errors created by cutting a 3D structure in two dimensions. In a generic sense, 3D imaging using any technique is superior to two-dimensional imaging for the purpose of calculating volumes and to a lesser extent EF. The latter is true because the errors in volume calculations tend to cancel out when expressed in a ratio to obtain EF.
Repeatability, precision, or test/retest variation, relates to the ability to obtain the same measurement on multiple tests when there has been no interval change of function. This parameter is often neglected but is extremely important in patient follow-up. Clearly a technique with a high degree of test/retest variation is unfavorable for follow-up applications. This is particularly a problem with two-dimensional imaging, because of the previously mentioned variations in cut planes in image data acquired at different times. Three-dimensional techniques are generally more repeatable, as are techniques that are independent of volumetric considerations such as global strain. Intraobserver and interobserver variability is often related to image quality. Although techniques with limited reproducibility may still be used with appropriate training, less variable methods have the potential attraction of operating at a high level of quality in less expert hands.
Combining these fundamental aspects of imaging technologies with the situations where LV imaging is required can provide some clues as to the most appropriate imaging choices. First, situations where accurate sequential follow-up is needed are most favored using 3D imaging, for example CMR, with 3D echocardiography being a good alternative. Although nuclear ventriculography has been used for this purpose, it may be less sensitive to small change. Subtle disturbances of systolic function may not be reflected by EF. In these situations, such as the follow-up of patients receiving cytotoxic chemotherapy, strain techniques could become the test of choice. Decisions requiring an accurate calculation of LV volumes or EF should be performed using an inherently 3D technique, such as MRI or 3D echocardiography. Such circumstances might include making decisions about device therapy in patients with heart failure, or when using quantitative methods to facilitate surgical decision making in patients with valvular heart disease. Assessment of regional wall motion is best performed using a high resolution technique, such as echocardiography or MRI. Contrast should be used with echocardiography whenever indicated by the failure to visualize more than two segments ; the benefit of contrast in the absence of suboptimal imaging is unproven and may be detrimental.
Conclusions
Despite its limitations, quantifying EF is accepted by the cardiology community. The evidence base for modern cardiology is so heavily based on this simple measurement that it is unlikely to disappear. The ubiquitous presence of cardiovascular diseases in currently aging societies mandates an inexpensive, widely available test that is able to provide hemodynamic assessment. Accordingly, echocardiography is likely to remain as the workhorse of LV functional assessment.
Left Ventricular Systolic Function: Basic Principles
- Zoran B. Popović, MD, PhD
- James D. Thomas, MD
Functional anatomy of the left ventricle
The left ventricle is a hollow muscular shell, capable of ejecting more than 100 mL of blood at over 200 mm Hg of pressure, and then filling with the same quantity of blood at less than 10 mm Hg in less than 100 msec during exercise. This becomes all the more remarkable when one realizes that the fundamental contractile unit of the cardiac myocyte, the sarcomere, is only capable of shortening by about 13%, whereas the ventricle shortens in length and circumference by about 20% with over 40% radial thickening, leading to the ejection of more than 60% of the end-diastolic volume. The transduction of this small degree of sarcomere shortening into an ejection fraction greater than 60% is only possible because of a very specific counterwoven double-helical arrangement of muscle fibers.
Before discussing the actual anatomy, consider an alternative, simpler arrangement consisting only of fibers coursing circumferentially in the cavity. The sarcomeres generate force most efficiently in a narrow range around 13%, but as shown in Figure 28.1 , to reduce the cross-sectional area by 60% with an incompressible material like the myocardium requires fiber shortening of approximately 15% at the midwall (near optimal for the sarcomere). But at the epicardium, there is only 7% shortening, which is too little for optimal force generation. Furthermore, at the endocardium, conservation of mass requires the fibers to shorten by 26%, well beyond what a sarcomere can do because it requires inefficient crumpling of the fibers. To generate ejection with pressure requires an arrangement of myofibrils that allows each sarcomere to contribute optimally to contraction.

In reality, as Figure 28.2 demonstrates, the left ventricular myofibrils are arranged roughly in a left-handed helix in the epicardium and a right-handed helix in the endocardium, with a circumferential arrangement in the midwall. These are not discrete layers, but rather show a continuous gradation from roughly + 60 to − 60 degrees (relative to the circumferential midwall fibers) when moving from endocardium to epicardium. Although this helps to normalize sarcomeric shortening, a further feature is required. The fibers must be arranged in discrete sheets defined by perimysial collagen so that during systole, they can tilt inward, which permits much greater regional deformation but keeps sarcomere shortening in the optimal zone ( Fig. 28.3 ).


The counterwoven helical architecture contributes to ventricular torsion, the wringing motion that helps to eject blood while also storing energy in the interstitium and titin, the sarcomeric molecular spring. The stored energy is then released during diastole to assist in the low-pressure filling of the heart. Because they are farther from the ventricular centroid, the left-handed fibers in the epicardium have a mechanical advantage over the right-handed endocardial fibers. Therefore they can rotate the apex counterclockwise (as viewed in the typical short-axis echo plane) while pulling the base clockwise, generating about 13 to 15 degrees of twist at rest.
Left ventricular volume and its dynamic geometry
The left ventricle is a complex ellipsoid structure that is characterized by one major and two minor axes. The two minor axes, although similar in length, are not identical; the axis lying in the septolateral (horizontal) plane is shorter than the one lying in the intercommissural (vertical) plane, because the interventricular septum has a lower curvature. During cardiac contraction, after initially becoming more spherical, the LV becomes more elliptical at end systole. Hearts with systolic failure characteristically become more spherical. LV geometry is also influenced by RV pathology; septolateral dimensions usually decrease in the setting of RV pressure overload. These changes are relevant because flattening of the septum elicits profound changes in regional LV contractility from interference with the Starling mechanism.
Cardiac cycle
The cardiac cycle itself is one of the most complex physiologic phenomena in existence. It refers to the interplay between electrical and mechanical events in the heart that lead to sequential filling and emptying of various cardiac chambers. This interplay can be viewed as a sequence in time (time domain) or in a framework of pressure-volume loops of the individual cardiac chambers. We will limit discussion here to the LV cardiac cycle ( Fig. 28.4 ).

The contraction of the LV is initiated by an electrical impulse, which in a normal heart starts a depolarization wave from the base of the septum. Once the wave reaches the “superhighway” of the Purkinje conduction system, it moves rapidly toward the LV apex, engulfing the entire heart and reaching the posterior base of the left ventricle within the next 35 msec. Depolarization of the muscle does not immediately start mechanical contraction. An additional 10 to 15 msec are needed for the fibers to begin shortening. Thus the usual definition of end diastole is the nadir of the Q wave (beginning of the R wave) because it is assumed that, at this time, most of the cardiac tissue has not yet started to contract, even if it has been electrically activated. This time point precedes the closure of the mitral valve (assessed by either Doppler, M-mode echocardiography, or phonocardiography), although, when viewed with high temporal resolution echocardiography, closure of the mitral valve appears to be more of a process than a discrete phenomenon. The interval between the Q wave and mitral valve closure is called electromechanical delay . In the presence of a wide QRS complex, it is much more difficult to define end diastole, because in this situation the peak Q wave or the beginning of the R wave can precede the beginning of contraction by more than 100 msec. A typical way of overcoming this is to assume that the time point just before mitral valve closure is end diastole. Yet another way to define end-diastolic pressure (more relevant to the catheterization laboratory, but can also be applied to echocardiography) is the time when the first derivative of LV pressure over time (dP/dt) reaches 10% of maximum LV dP/dt. Regardless of its definition, the end-diastolic point closely corresponds to the lower right corner of the LV pressure-volume (PV) loop diagram ( Fig. 28.5 ).

Although the discussion on how to define end diastole may seem academic, it is crucially important whenever LV systolic function must be assessed, whether using familiar LV volumes or, especially, novel parameters such as strain. With the closure of the mitral valve (MV), LV pressure starts to rise. The LV then becomes more spherical, finally resulting in the opening of the aortic valve and the onset of ejection. The period between MV closure and aortic valve opening is the isovolumic contraction time (IVCT), whereas the period between the beginning of depolarization and aortic valve opening is the preejection period (PEP), which is the sum of the electromechanical delay and preejection period. Several physiologic events occur with aortic valve opening: the LV gradually becomes smaller and less spherical, and pressure does not increase as rapidly. LV dP/dt reaches its maximum (LV dP/dt max) almost simultaneously with aortic valve opening (see more later). The LV ejection period (defined as the time between aortic valve opening and closure) is classically characterized by two parts, which are rapid (early) and slow (late) ejection; although the differentiation between these two periods is not as clear in the setting of high afterload or low contractility. Interestingly, most of the blood volume is ejected while LV pressure is relatively constant (see PV loop diagram in Fig. 28.5 ). As we near the end of ejection, a relatively rapid pressure drop occurs. In the PV loop diagram, this is marked as the left upper point on the diagram, defining physiologic end systole (in mathematical terms, this is the maximum of the pressure-to-volume ratio). This time point usually precedes aortic valve closure by 15 to 30 msec, except in some pathologic states (e.g., mitral regurgitation), and marks the beginning of relaxation. With aortic valve closure, the rate of pressure drop increases rapidly, leading to the occurrence of peak negative dP/dt (dP/dt min), a marker of relaxation (see next section). LV pressure continues to drop, albeit more slowly, until the mitral valve opens, which marks the end of the isovolumic relaxation time (IVRT) period. This pressure drop during IVRT follows an approximately exponential decay curve characterized by the time constant of isovolumic pressure decay (see next section). With mitral valve opening, there is an interplay with continued relaxation and early filling of the left ventricle, which leads first to a short period of pressure drop up to minimum LV diastolic pressure (with an increase in volume and thus, instantaneously, “negative” compliance). After minimum LV diastolic pressure, there follows a relatively long period of continuous pressure increase, augmented by atrial contraction, and culminates with mitral valve closure and the end of this LV filling period.
Determinants of LV performance
The basis of LV performance is summed up by the Harvey principle of continuous (closed-loop) circulation of the blood and the Starling mechanism of cardiac output regulated by its preload (i.e., input), enabling the heart to work as a servomotor. Determinants of LV performance are usually identified as preload, afterload, contractility (inotropy), and diastolic function (lusitropy and chamber compliance; see later); however, not all of these determinants are completely independent.
LV preload refers to initial sarcomere length, and it is linked to cardiac performance through the Starling mechanism. In summary, an increase in preload (i.e., the amount of blood coming into the heart) leads to an increase in the force generated by sarcomeres, which in turn leads to increased LV pressure, power, and work. The area of the pressure-volume loop quantifies the amount of work generated in each heart stroke. There are two ways in which stroke work may be increased. The first is by augmenting the difference between diastolic and systolic pressures, and the second is by increasing the difference between diastolic and systolic volumes. Both mechanisms may contribute, as well. Because we cannot measure sarcomere length, preload is quantified by end-diastolic volume. As a surrogate, one can use pulmonary capillary wedge pressure, mean left atrial pressure, LV end-diastolic pressure, or LV mean diastolic pressure, all of them strongly correlated but with somewhat different physiologic determinants.
LV afterload is the amount of resistance that the heart encounters during ejection. It is very important to understand that afterload under normal condition is “good”; most of the vital organs need some level of blood pressure so they can operate their autoregulatory blood supply systems. If blood pressures were in the range of 50 mm Hg, no matter how many liters of blood were pumped through the circulatory system, the brain, kidneys, and heart would not be able to extract sufficient oxygen for adequate function. In this regard, septic shock can be considered a situation in which afterload is inadequate. However, an afterload that is too high leads to a decrease in stroke volume because pressure and flow are linked within the pressure-volume loop. Afterload may be separated into two components, oscillatory and static. The static component represents capillary resistance and can be quantified simply by a systemic vascular resistance equation as cardiac output divided by the difference between mean systolic pressure and central venous pressure. The oscillatory component reflects elasticity of the aorta and big vessels, and it is defined by the interplay between arterial pressure and flow that can only be quantified using complex computational methods. Yet another measure, which theoretically represents the sum of both components, is arterial elastance, defined as the ratio between end-systolic pressure and stroke volume.
We have already mentioned that LV force, power, and work are determined by preload, augmenting with increased preload. So how do we then define LV contractility? Although there are several possible ways, potentially the easiest one is that LV contractility represents the increase in the amount of force, power, or work per unit increase in preload (i.e., end-diastolic volume). Experimental work has shown that the relationship between cardiac performance parameters and end-diastolic volume is nearly linear, with the slope of this relationship representing contractility ( Fig. 28.6 ). When dP/dt max is used as the parameter of interest, the name of the slope is elastance derivative (dE/dt). When stroke work is used, the name is preload recruitable stroke work. Finally, when LV power is used, the name of the relationship is preload-adjusted maximal power. Yet another way to understand these complex concepts is through the assessment of end-systolic pressure volume relationships. In brief, if a sudden decrease in preload occurs, blood pressure drops precipitously, as the end-systolic volume decreases and afterload is suddenly reduced by the blood pressure drop. The relationship between blood pressure and end-systolic volume decrease is again linear, with the slope of this relationship representing end-systolic elastance. Of note, there are several other closely related concepts, such as time-varying maximum elastance, which we will not cover because they are complex and infrequently used, even in the physiology community. Elastance is difficult to understand in the context of the Starling law. One way to gain insight is to interpret this concept as a measure of resistance of the LV to increase its size with increasing systolic blood pressure. One might imagine what would happen if the aorta were constricted. A heart with high elastance will maintain initial end-systolic volume, whereas one with low elastance will increase its end-systolic volume.

This leads to a final method for assessing contractility, which is the relationship between wall stress and ejection fraction (or fractional shortening). Wall stress is a force acting in a given direction per unit area, and it represents a measure of a force that opposes myocardial fiber contraction, or in other words, afterload. In addition to intracavitary pressure, two other factors influence stress: LV cavity size and wall thickness. For a same pressure, force (stress) will be higher in a larger ventricle because the ventricular surface over which intracavitary pressure is acting is larger. Inversely, increased wall thickness decreases stress because it distributes force over a larger linear dimension. The final concept is similar to the concept of elastance: hearts with high contractility can have a high ejection fraction despite high stress, but the opposite will be true for the failing heart. Plotting wall stress on the x -axis and ejection fraction on the y -axis in Cartesian coordinates enables comparison with previously ascertained normal stress–ejection fraction values without the need for further preload manipulation ( Fig. 28.7 ).

Of note, in clinical cardiology, parameters that require preload manipulation in their calculation are seldom used. A key reason is that one needs to know the so-called V 0 , which is the minimum LV volume that elicits a cardiac response. Although there are several methods to estimate V 0 , these are usually taken as rough estimates and not as actual measures. Ejection fraction–wall stress relationships are also almost never explicitly used in modern cardiology for adult patients because they often exhibit low sensitivity to depressed contractility. However, one point is critical: any echocardiographic examination is diminished in value if the blood pressure is not recorded during the examination.
Response to exercise
Exercise elicits a complex and powerful response of the cardiovascular system brought about sympathetic activation and multiple autoregulatory cardiac mechanisms. Muscle mobilization leads to decreased venous capacitance and increased preload. In other words, muscle contraction pumps out blood out of the limb vasculature into the central circulation. This in turn leads to reflex tachycardia (Bainbridge reflex) and increased contractility through Bowditch (force-frequency) phenomenon. There is a decrease of peripheral resistance caused by arteriolar vasodilation in muscles, leading to decreased afterload and increased cardiac output. At the same time, there is sympathetic activation that further leads to increased contractility, heart rate, and relaxation rate. All of these phenomena lead to the heart delivering greater cardiac output in a hemodynamically optimized (less afterload) environment. These phenomena lead to moderate increases in blood pressure, decreases in end-systolic volume (larger than the decrease in end-diastolic volume), mild increases in stroke volume, and an overall increase in the cardiac output, mostly because of increased heart rate. Heart rate increase is not a necessary mechanism because cardiac output increases even in the setting of a fixed heart rate; however, the total increase in cardiac output is compromised if heart rate does not increase. Cardiac exercise also leads to a mild increase in LV filling pressures. Of interest is that, although changes in EF and strain are minimal, there is a dramatic increase in strain rate, both positive and negative.
Global Left Ventricular Systolic Function
- Alex Pui-Wai Lee, MBChB
- Cheuk-Man Yu, MD
Assessment of left ventricular (LV) systolic function should be part of almost all echocardiographic examinations. This chapter discusses various traditional and newer approaches to the quantification of LV global systolic function.
Echocardiographic methods used to assess LV global systolic function
M-Mode Echocardiography
M-mode echocardiography records the motion of cardiac structures in one dimension. Linear measurements of LV dimensions (LVDs) are made by positioning the cursor through the LV minor axis at the level of mitral leaflet tips in the parasternal long-axis view. Fractional shortening (FS) and ejection fraction (EF) can then be calculated with geometric assumptions.
Two-Dimensional Echocardiography
Two-dimensional echocardiography (2DE) remains the primary tool for evaluation of LV systolic function. Endocardial border motion and wall thickening can be visualized for “eye-balling” of LV function. Quantitatively, volumetric measurements are obtained by tracing the endocardial border in apical four- and two-chamber views in end diastole and end systole using the biplane method of discs (modified Simpson rule) ( Fig. 29.1 ). The LV is mathematically divided along its long axis into a series of discs of equal height. Individual disc volume is calculated as height × disc area (assuming circular discs). LV volume is then represented by the sum of disc volumes.

Three-Dimensional Echocardiography
Three-dimensional echocardiography (3DE) provides volumetric measurements without geometric assumptions. Typically, a full-volume data set of the LV obtained from the apical window is analyzed by either tomographic or surface rendering techniques. In the tomographic approach, reconstruction of cut planes from a 3D data set ensures no foreshortening when calculating the left ventricular ejection fraction (LVEF) based on the Simpson rule. Alternatively, a surface-rendered cavity cast of LV can be created to allow computation of LV volume from voxel counts, and its change over time is tracked to calculate EF and stroke volume ( Fig. 29.2 ). 3DE has been clearly demonstrated to provide more accurate and reproducible measurements of LV volumes than 2DE, and is comparable to magnetic resonance imaging (MRI).

Doppler Echocardiography
Doppler echocardiography provides hemodynamic information. Calculation of stroke volume (SV) uses the hydraulic orifice formula: SV = left ventricular outflow tract (LVOT) velocity time integral × LVOT area (assuming a circular LVOT). Without aortic regurgitation, SV reflects blood volume ejected per beat and is the hemodynamic product of LV contraction.
Tissue Doppler Imaging
Tissue Doppler imaging (TDI) uses the Doppler principles to measure velocities of tissue movements. A wall filter is used to distinguish between signals originating from moving tissue (high amplitude, low velocities) and blood flow (low amplitude, high velocities). The systolic velocity of the mitral annulus (S′) correlates well with LVEF and SV, with important prognostic implications. Strain rate and strain (primarily longitudinal component) can also be derived from TDI.
Speckle Tracking Echocardiography
Speckle tracking echocardiography (STE) is a novel technique used to evaluate cardiac mechanics. The speckles seen in 2D gray-scale images result from interference of ultrasound scattered from structures smaller than the ultrasound wavelength. With STE, kernels of speckles can be tracked from frame to frame by block matching, providing displacement information, from which myocardial strain and SR can be derived. Strain measurements by 2D STE varies with myocardial regions, age, gender and echocardiographic vendors in healthy adults.
Global LV systolic functional parameters
Fractional Shortening and Ejection Fraction
Fractional shortening (FS) is calculated from linear measurements of LVD from M-mode or 2D images:
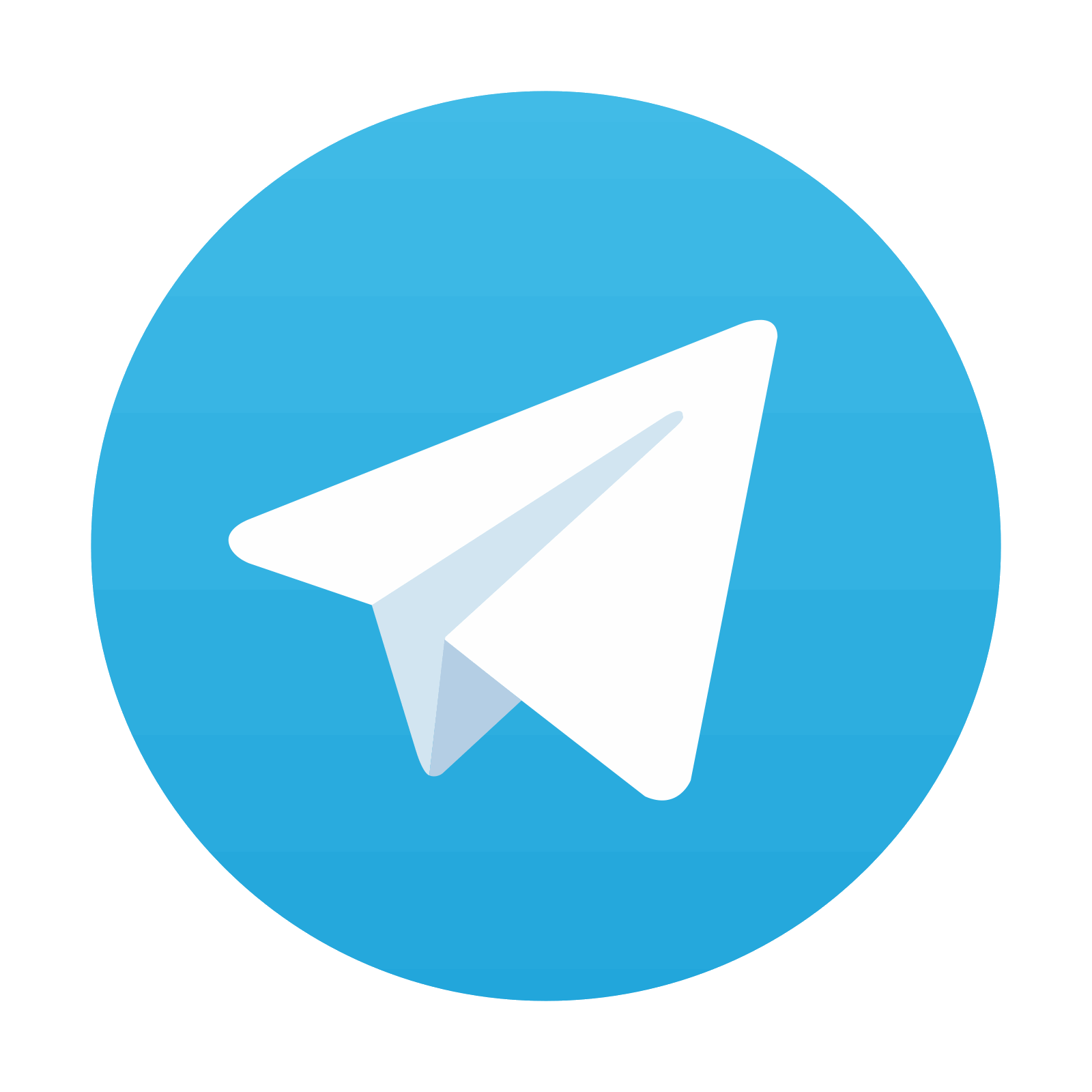
Stay updated, free articles. Join our Telegram channel
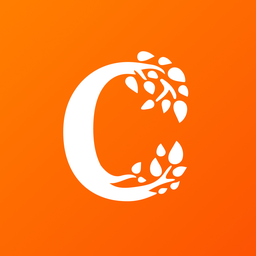
Full access? Get Clinical Tree
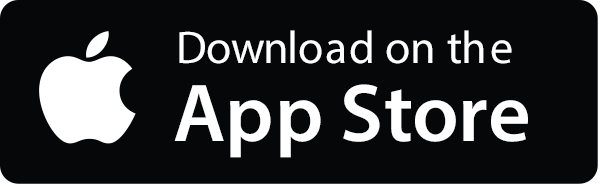
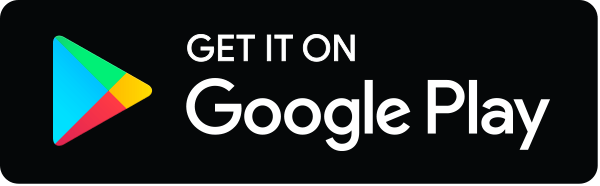