Introduction
Catastrophic air pollution events in the Meuse Valley of Belgium (1930), Donora, PA (1948), and London (1952) arose when air stagnation caused high levels of ambient pollutants, especially sulfur dioxide and particles. In each case, there was a marked increase in respiratory symptoms and mortality. These “fog disasters” provided incontrovertible evidence that air pollution can have immediate lethal consequences. Since this time, scientific investigations have uncovered myriad adverse respiratory health effects of air pollution, ranging from asthma exacerbation to respiratory mortality.
The respiratory system, because it is the portal of entry for air pollutants, suffers the major consequences of air pollution. This chapter reviews the key air pollutants, which are termed criteria pollutants by the U.S. Environmental Protection Agency (EPA), and how they affect the airways and lungs ( Table 74-1 ). Both indoor and outdoor air pollution are considered. Respiratory health conditions that are most strongly linked with air pollution are reviewed.
Pollutant | Standard * | Averaging Period |
---|---|---|
Particulate matter < 10 µm (PM 10 ) | 150 µg/m 3 | 24 hr |
Particulate matter < 2.5 µm (PM 2.5 ) | 12 µg/m 3 | 1 yr |
35 µg/m 3 | 24 hr | |
Sulfur dioxide | 75 ppb (214 µg/m 3 ) | 1 hr |
500 ppb (1430 µg/m 3 ) | 3 hr | |
Nitrogen dioxide | 100 ppb (188 µg/m 3 ) | 1 hr |
53 ppb (100 µg/m 3 ) | 1 yr | |
Ozone | 75 ppb (100 µg/m 3 ) | 8 hr |
Lead | 0.15 µg/m 3 | Rolling 3-mo average |
Carbon monoxide | 9 ppm (10 mg/m 3 ) | 8 hr |
35 ppm (40 mg/m 3 ) | 1 hr |
* Where two standards are listed, the first is the primary air quality standard set by the U.S. Environmental Protection Agency to protect health. The second standard listed is the so-called secondary standard, which is set to protect welfare.
Air Pollutants
Pollutants—What They are and Why They Matter
Air pollution involves the contamination of the atmosphere by chemicals, particles, or biologic materials that causes human morbidity and mortality, damages other living organisms such as plants, or adversely affects the natural environment such as by reducing visibility. Pollutants can be emitted from mobile sources (e.g., vehicles), stationary sources (e.g., factories, power plants, refineries), and indoor sources (e.g., building materials, gas stoves, passive smoking, cleaning products).
Toxic air pollutants (air toxics) are a subset of air pollutants that may cause cancer or other serious health effects such as birth defects. These substances, such as benzene and cadmium, produce primarily nonrespiratory effects despite entering the body via the respiratory tract.
The major types of air pollutants affecting the respiratory system are particulate matter (PM), sulfur dioxide (SO 2 ), nitrogen dioxide (NO 2 ) and ozone (O 3 ) ( Table 74-2 ). The principal sources of these pollutants are mobile and stationary sources. Ozone is a secondary pollutant that arises from the action of sunlight on nitrogen oxides and hydrocarbons present in motor vehicle exhaust. It is a major component of photochemical pollution or “smog.” The respiratory health effects of these pollutants are considered later in the chapter. Two other air pollutants, carbon monoxide (CO) and lead, which also enter via the respiratory tract, have primarily nonpulmonary toxicity and are not considered further in this chapter.
Pollutant | Outdoor Sources | Indoor Sources | Adverse Effects |
---|---|---|---|
Particulate matter | Motor vehicle exhaust, power plants | Tobacco and wood smoke | Exacerbations of asthma and chronic obstructive pulmonary disease, increased cardiopulmonary mortality |
Sulfur oxides | Power plants, oil refineries, smelters | Kerosene space heaters | Bronchoconstriction |
Nitrogen oxides | Motor vehicle exhaust, power plants, oil refineries | Gas stoves and furnaces, kerosene space heaters | Airway injury (respiratory bronchiolitis), impaired lung defenses, enhanced response to allergens |
Ozone | Motor vehicle exhaust | Aircraft cabins, welding, copiers, ozone generators | Airway injury (respiratory bronchiolitis), decreased lung function, exacerbations of asthma, enhanced response to allergen |
Radon | None | Residential basements | Lung cancer |
Polycyclic aromatic hydrocarbons | Diesel exhaust | Tobacco smoke | Lung cancer |
Indoor air quality has assumed increasing importance. As levels of outdoor ambient air pollution have decreased in many areas throughout the United States, the relative impact of indoor air pollution has increased. Moreover, North American residents spend the majority of their time indoors, increasing their exposure to indoor air pollutants. At the same time, changes in home and office building construction have resulted in lower air exchange rates, increasing the levels of pollutants emitted indoors. In the developing world, indoor air pollution due to biomass smoke from domestic cooking and heating is a major source of morbidity and mortality.
General Properties, Sources, and Distribution of Pollutants
PM, sulfur oxides, NO 2 , and O 3 are the most widely encountered pollutants that cause adverse pulmonary health effects.
PM is an important pollutant. In the western United States and many other areas of the world, particulate pollution is not driven by the combustion of sulfur-containing fuels. Atmospheric particulate air pollution arises from a variety of sources, including both natural (e.g., sea spray, windblown dust) and synthetic (e.g., power plants, motor vehicles) sources. Moreover, particulate material that enters the atmosphere can be primary (particles emitted directly) or secondary (particles formed by complicated chemical reactions happening in the atmosphere and involving gas-phase precursors such as SO 2 and nitrogen oxides). In North America, secondary particles comprise most of the fine-particle pollution.
In epidemiologic studies, researchers have defined the size of particles in terms of aerodynamic diameter because most particles have irregular shapes with geometric diameters that cannot be easily measured. The aerodynamic diameter is the diameter of a perfect sphere of unit density (1 g/mL) that has the same aerodynamic properties (e.g., settling velocity) as the particle in question. Larger particles are filtered in the nose and throat, but particles less than 10 µm in diameter (i.e., PM 10 ) can be deposited in the respiratory tract. Fine particles, which are less than 2.5 µm in diameter (PM 2.5 ), penetrate to the alveoli, and ultrafine particles (PM 0.1 ) can pass through the alveoli and spread to other organs.
Size also determines how far particles travel and persist in the environment. Fine particles can travel for long distances and remain in the atmosphere for days to weeks; “coarse” particles (>2.5 to 10 µm) travel for rather short distances and have atmospheric half-lives of minutes to hours. Ultrafine particles are typically generated from combustion processes and have short atmospheric half-lives. For example, the concentration of ultrafine particles emitted from motor vehicles on a major roadway has a rapid falloff with distance from the roadway; the concentration is back down to the background level at or beyond 300 m.
Fine particles (PM 2.5 ) are composed mainly of varying amounts of water and several major components (sulfates, acids, nitrates, elemental carbon, organic carbon, and trace metals) depending on sources. They are directly emitted during combustion but are also formed from gases by nucleation, condensation, or liquid-phase reactions. Coarse particles (PM 10-2.5 ) are composed primarily of crustal (rock, soil), biologic (pollen, spores), and industrial components; they are formed primarily by mechanical processes that produce small particles from larger particles.
SO 2 is produced by the combustion of sulfur contained in fossil fuels such as coal and crude oil. The major sources of SO 2 pollution are power plants, oil refineries, smelters, and paper pulp mills. In the United States, SO 2 levels are generally higher in the Northeast and Midwest because sulfur-containing coal is used in power plants. Sulfur dioxide itself is a clear, highly water-soluble gas, so it is effectively absorbed by the mucosal surfaces of the upper airways. A small proportion of inhaled SO 2 reaches the distal regions of the lungs, but susceptible persons such as those with asthma may still suffer adverse respiratory health effects. The SO 2 released into the atmosphere does not, however, remain uniquely in the form of a gas; rather, it undergoes chemical reactions with water, trace metals, and other pollutants to form particulate aerosols. The nature of particles generated from sulfur-containing fuels varies geographically, but sulfuric acid and various metallic, acidic, and ammonium sulfates are commonly present. The mixture of sulfur oxides and small particles may be blown great distances from its source, undergoing continuous transformation from gas to particle phase and ultimately becoming “acid rain” capable of profoundly affecting flora and fauna.
NO 2 is rapidly generated from nitrogen oxides, which are produced whenever there is combustion, and motor vehicles and power plants are the major sources of this pollutant. The areas with the highest concentrations of NO 2 tend to be the downtown cores of large cities where there are major roadways and traffic congestion. Because NO 2 tracks well with traffic emissions, it is often considered a marker of traffic-related air pollution in epidemiologic studies. NO 2 reacts with oxygen to generate O 3 and nitric oxide, but this is a bidirectional reaction so that O 3 levels tend to be lower in areas where NO 2 is concentrated. Thus, O 3 levels tend to be lower in neighborhoods adjacent to major roadways.
O 3 arises primarily from the action of sunlight on emissions from internal combustion engines of motor vehicles. The most important of these emissions are unburned hydrocarbons (so-called volatile organic compunds or VOCs) and nitrogen oxides. In the atmosphere farther away from traffic sources, ultraviolet irradiation of the mixture of VOCs and NO 2 results in a complex series of chemical reactions, producing O 3 , alkyl nitrates, peroxyacyl nitrates, alcohols, ethers, acids, peroxyacids, and other organic and inorganic compounds that exist in both gas and particulate aerosol phases. This mixture of pollutants typifies the “smog” found in areas with large numbers of automobiles and abundant sunlight, such as the Los Angeles basin.
Because O 3 and NO 2 are the gases present in the highest concentration in smog and they clearly cause toxic effects in animals and humans, they have been the oxidant pollutants most extensively studied. Both gases are relatively insoluble and poorly absorbed by the upper airways. A high proportion of the inhaled dose therefore reaches the peripheral portion of the lungs and can cause injury at any site from the upper airways to the alveoli.
Outdoor urban air contains a number of known lung carcinogens, including polycyclic aromatic hydrocarbons (PAHs), n -nitroso compounds, and asbestos. The concentrations of these carcinogens in ambient air, however, are quite low. Diesel exhaust, in particular, contains particles with PAHs as constituents. Epidemiologic studies have provided consistent evidence of an excess risk of lung cancer in workers exposed to diesel exhaust; it is listed by the International Agency on Cancer Research as a known human carcinogen. Several large population-based epidemiologic studies have also linked lung cancer to long-term exposure to PM, SO 2 , and O 3 pollution.
Although most attention has been given to pollutants present in outdoor air, it is now apparent that elevated concentrations of airborne contaminants are common inside homes, public buildings, and other indoor micro-environments. In developed countries, there are point sources of pollution indoors, such as secondhand tobacco smoke and gas stove use, that greatly increase the concentration of pollutants compared with outdoor air. In developing countries, biomass fuels are still burned indoors in poorly efficient stoves for cooking and heating. Biomass smoke is a mixture of gases and PM that is similar to tobacco smoke with the exception that it does not contain nicotine. Both indoor and outdoor pollution must be considered when assessing the total health effects of air pollution.
Mechanisms of Defense Against Air Pollution–Related Respiratory Effects
The respiratory system, which encounters myriad particles and gases daily, has evolved an effective defense system for removing impurities from inhaled air. The various mechanisms of defense are interrelated and work in a coordinated manner. A perturbation of any defense mechanism, whether because of congenital deficiency, disease, or the effect of an inhaled pollutant itself, may result in the breakdown of the coordinated defense system and the development of disease.
Deposition of and Clearance of Particles (see Chapter 11 )
The size of particles determines their site of deposition in the respiratory tract. Large particles (diameter > 10 µm) are efficiently removed in the nose by simple filtration via the cilia. Those particles that are not removed by filtration will largely be deposited by a process termed impaction. The pathway through the nose, mouth, and branching airways is tortuous. Because inhaled particles cannot easily change direction to follow the abrupt changes in the pathway of airflow, they impact on the mucosa of the upper and lower respiratory tract. Airway bifurcations are especially prone to particle impaction. The complementary mechanisms of filtration and impaction are efficient, so few particles more than 10 µm reach the lower respiratory tract.
Smaller particles are less affected by filtration and impaction; the sites of their deposition are more determined by the processes of sedimentation and diffusion. Sedimentation is the tendency for particles to fall at a constant rate under the influence of gravity. It is strongly influenced by particle density, particle diameter, and the viscosity of the surrounding gas. As air moves deeper into the lung, the airflow rate decreases and particles fall out of the air stream under the influence of gravity. Sedimentation is the predominant mechanism for deposition of particles of intermediate size (0.5 to 3.0 µm) in the smaller bronchi, bronchioles, and alveoli.
The smallest particles (<0.5 µm), however, are deposited by the mechanism of diffusion. These particles have random (Brownian) motion and will impact the airway mucosa in the terminal bronchioles and alveoli, removing them from the stream of inspired air.
The pattern of breathing can influence the deposition of particles. A higher respiratory rate increases the velocity of airflow, promoting impaction of particles in the more proximal airways. Conversely, increased tidal volume results in deeper lung penetration by particles; when the respiratory rate is slow, there is more time for diffusion and sedimentation to deposit particles in the distal airways and alveoli.
After particles are deposited in the tracheobronchial tree, cough and mucociliary clearance are the most important mechanisms of defense. Cough is most effective for clearing particles that are deposited in the larger, more central airways. It is provoked by stimulation of afferent nerves that are most densely present in the mucosa of the larynx and branching points of the tracheobrochial tree, at which particles are most likely to be deposited.
The “mucociliary escalator” is a critical system for clearing particles that are deposited in the tracheobronchial tree; it operates over the respiratory tract from the proximal trachea to the terminal bronchioles. The mucus blanket is the product of secretion by goblet cells and serous cells of the epithelium and by the mucus cells and serous cells of the submucosal glands. The mucus blanket is propelled mouthward by the coordinated beating of epithelial cell cilia. The effective removal of particles by the mucociliary system thus requires secretion of glycoproteins by specialized secretory cells, maintenance of liquefaction by the transport of water and solute by airway epithelial cells, and coordinated function of airway cilia. Impairment of any of these functions, whether by congenital or acquired diseases affecting mucus or water secretion (e.g., cystic fibrosis, chronic bronchitis) or ciliary function (e.g., dysmotile cilia syndrome), leads to longer residence of particles in the airway and a greater likelihood of adverse health effects.
Some particles will be deposited in the distal airway and alveoli, beyond the reach of the mucociliary escalator. The central mechanism for clearing these particles involves the alveolar macrophage, which phagocytoses particles that are deposited in the gas-exchanging parts of the lung and either digests them or migrates to the respiratory bronchiole and ascends the mucociliary escalator. A smaller number of particle-laden macrophages migrate to peribronchial or perivascular connective tissue, which is a slow method of clearance that takes many weeks. Because macrophages are the primary phagocyte for inhaled PM, the carbon content of macrophages has been used as a biomarker of exposure to particulate pollution in epidemiologic studies.
Gases—Deposition and Damage are Functions of Solubility
For gaseous pollutants such as O 3 , NO 2 , and SO 2 , the site of deposition in the respiratory tract is largely a function of water solubility. For highly soluble gases, the nose serves an important defensive function because the air inhaled transnasally is passed over the large, irregular surface of the turbinates. The promotion of turbulent flow prolongs contact with the mucosal surface, increasing adsorption. Under conditions of quiet breathing, when most air is inspired transnasally, the concentration of SO 2 that reaches the glottis is less than 2% of the concentration inspired at the nose. The more rapid the inspiratory flow rate, the smaller the proportion of SO 2 removed. Furthermore, the mouth is substantially less efficient at absorbing gases than is the nose, and the proportion of air inhaled through the mouth increases as the ventilatory demands of exercise rise. Therefore, the dose of a soluble pollutant delivered to the lower airways is increased by exercise for three reasons: the inspiratory flow is higher, the proportion inhaled through the mouth is increased, and the total quantity inhaled over time is greater. The importance of the level of ventilation, which depends on the level of exercise, and of the oral-nasal distribution of breathing has perhaps best been shown in studies of the bronchoconstrictor response to SO 2 , in which the responses are greatest during oral breathing and smallest during nasal breathing. In addition, people with obstructive nasal disease also appear to be at greater risk for adverse respiratory effects of soluble pollutant gases.
Oxidative Stress
Air pollution, especially particles and O 3 , can increase the oxidative burden to the lung. To minimize oxidant damage to biologic molecules, the human lung has an integrated antioxidant system of enzymatic and expendable soluble antioxidants. This system includes several antioxidant defense mechanisms that detoxify reactive products or convert them to products that are quenched by other antioxidants. If the oxidant burden is sufficiently great, the reactive species may overwhelm or inactivate the antioxidant system. An imbalance between the production of reactive oxygen species (ROS) and reactive nitrogen species and antioxidant capacity leads to a state of “oxidative stress” that contributes to the pathogenesis of several respiratory diseases.
When imbalance exists, the excess oxygen species induce a cellular stress response that activates a number of the redox-sensitive signaling cascades. This initial response is mediated in large part through the transcription factor nuclear factor erythroid 2–related factor 2, which leads to transcriptional activation of more than 200 antioxidant and detoxification enzymes that are collectively known as the phase 2 response. Examples of phase 2 enzymes include heme oxygenase 1, glutathione- S -transferase isoenzymes, NADPH quinone oxidoreductase, catalase, superoxide dismutase, and glutathione peroxidase. If phase 2 responses fail to prevent a further increase in ROS production, major cellular components, including membrane lipids, protein, carbohydrates, and DNA, will be damaged and inflammation and tissue damage may ensue.
Oxidative stress-induced proinflammatory effects are mediated by the redox-sensitive mitogen-activated protein kinase and nuclear factor-KB cascades that are responsible for the expression of cytokines, chemokines, and adhesion molecules. Cytotoxic effects are associated with mitochondrial membrane damage, which leads to release of factors that induce apoptosis of lung cells. Pathophysiologic consequences of pollutant-induced oxidant injury include airway inflammation leading to clinical exacerbation of asthma, increased susceptibility to infection, enhanced allergic responses, and, if repetitive or chronic, airway remodeling.
Methods of Studying the Health Effects of Air Pollution
In order to develop sound public policy aimed at protecting the public from the adverse health effects of pollutants, scientific studies are necessary both to elucidate the health effects of various pollutants and to define the threshold level at which the risk substantively increases. The scientific basis of public policy is based on several lines of evidence: epidemiologic studies of the association between air pollution and disease in the population, controlled human exposure studies conducted in the laboratory, studies of the effects of pollutants on lung structure and function in animals, and in vitro studies of toxicity. Each of these study designs has advantages and disadvantages, and none alone can provide irrefutable proof of harmful effects from a particular pollutant under naturalistic conditions of exposure ( Table 74-3 ). Air quality standards are based, therefore, on the coherence of evidence provided by epidemiologic, clinical, animal, and in vitro research and are set to provide a margin of safety to protect susceptible subgroups of the general population.
Method | Strengths | Weaknesses |
---|---|---|
Epidemiologic study |
|
|
Controlled human exposure study |
|
|
Animal study |
|
|
In vitro study |
|
|
Epidemiologic Studies
Epidemiologic studies have a major role in the evaluation of air pollution–related health effects because they involve “real-world” exposures of human subjects. The strengths of epidemiologic studies include the ability to evaluate a wide array of health effects and representative sampling of the larger population. These studies, however, have to address issues of confounding and other sources of bias. Moreover, epidemiologic studies, although they play a key role in support for regulatory intervention, do not provide mechanistic data; human, animal, and cellular toxicologic data are required to understand mechanistic pathways.
For each putative pollution-related health effect, evidence must be compiled from published literature, synthesized, and evaluated for the likelihood of a causal effect. Typically, the guidelines for evaluating whether a particular pollutant (e.g., PM) causes a specific respiratory disease (e.g., chronic obstructive pulmonary disease [COPD]) are those introduced by Sir Austin Bradford Hill and used by the U.S. Surgeon General beginning in 1964. These include strength of the association, consistency of the association, specificity of the association, temporal relationship, coherence, biologic plausibility, and exposure-response gradient. Using this approach to evaluate the strength of the evidence provides a sound basis for regulatory decisions.
The issue of confounding must be addressed in epidemiologic studies of air pollution–related health effects. It is often difficult to be sure that all confounders (e.g., cigarette smoking, occupational exposures, socioeconomic status) have been identified and controlled. The use of daily time-series studies that correlate daily average air pollution levels (e.g., PM 2.5 ) with daily counts of a health outcome (e.g., deaths) is one method that provides good control of confounders that do not change over relatively short time periods.
Another difficulty with epidemiologic studies is that most outdoor environments include a complex mixture of pollutants. Assessing the effects of individual pollutants, one by one, might miss the effects of the mixture. A related issue is “multicollinearity,” which arises because air pollutant concentrations are correlated with one another, making it difficult to parse out the individual health effects of each pollutant. Sophisticated statistical modeling attempts are used to address these potentially confounding issues, but they remain challenges to valid inferences.
Another major challenge to air pollution epidemiologic studies is exposure assessment. Many studies estimate individual exposure by mapping each individual subject’s home (and sometimes schools or workplaces) relative to stationary pollution monitors. Recent advances have also allowed investigators to conduct personal monitoring of exposure to specific pollutants, but expense and other logistical considerations may make this infeasible. Choosing the metric of exposure that is most relevant to disease causation requires careful consideration. With O 3 , for example, the 8-hour average from 10 am to 6 pm may be most appropriate because the EPA air quality standard uses an 8-hour averaging time and these hours reflect the impact of photochemistry on O 3 precursors emitted from motor vehicles during the morning commute. In contrast, shorter peak exposures would be more appropriate for assessing the effect of SO 2 on exacerbations of asthma. Whether a time “lag” between onset of exposure to the pollutant and onset of the health outcome (e.g., one or several days) should be considered is also an important question.
Controlled Human Exposure Research
Experimental studies expose human subjects to pollutants under carefully controlled conditions. Such studies can rigorously evaluate the impact of a single pollutant or a mixture such as diesel exhaust on acute responses such as lung function or airway inflammation. In addition to documenting the acute health effects of pollution, controlled human exposure studies can evaluate the minimal concentration of a pollutant that causes reduction in lung function or other acute responses. These studies can also evaluate the effects of pollutants on susceptible subgroups of the population, such as on adults with asthma. Moreover, measurement of biomarkers of inflammation, oxidative stress, and other pathophysiologic processes can provide mechanistic insights.
A major advantage of controlled human exposure studies is the (near) elimination of confounding and bias because exposures are experimentally induced and carefully controlled. Because these studies evaluate acute effects, however, they cannot, by themselves, be easily extrapolated to the effects of more chronic or recurrent exposure. The finding that a brief exposure to O 3 causes acute decrements in lung function does not, for example, necessarily indicate that prolonged or repeated exposure will lead to the development of COPD. However, controlled human exposure studies, when combined with epidemiologic and animal toxicologic studies, can provide important data for establishing the causal effect of pollution on a respiratory health outcome.
Animal Research
Animal studies offer the most effective way to investigate the impact of air pollution on lung structure. In addition, animal studies permit assessment of the effects of a range of concentrations so that a dose-response curve can be constructed for outcomes ranging from the first measurable change in lung function or structure until death. Animal studies also afford a unique opportunity to dissect the pathogenetic mechanisms of pollution-related health effects.
The major limitation of animal studies is the large interspecies variations in response to pollutants. As such, applicability of animal studies to humans, especially for dose-response relationships, may not always be clear. Rodents, for example, can tolerate relatively high levels of O 3 without developing structural injury to the lungs, whereas rhesus monkeys develop injury at levels approaching ambient concentrations. Moreover, common human lung diseases, such as asthma and cystic fibrosis, have not been fully reproduced in animals, precluding study of these susceptible subgroups for pollution-related effects.
In Vitro Research
Exposure of cell cultures to specific air pollutants has helped to elucidate the mechanisms of lung injury, inflammation, and other adverse respiratory health effects. Specifically, cell culture systems have been used to study the impact of particulate and other air pollutants on inflammatory cytokines, oxidative stress, DNA damage, and cytotoxicity. Cultures of bronchial and alveolar epithelial cells, monocytes, dendritic cells, and other cell lines have been useful for studying the mechanisms of air pollution on the respiratory system.
Air Pollution Studies—Putting It All Together
In sum, there is no single study design or discipline that can establish the health effects of air pollution and provide the basis for regulation. Epidemiologic studies are required because they evaluate the impact of real-world air pollution on respiratory health outcomes among the general population. The limitations of epidemiologic studies usually mean that coherence of epidemiologic findings with those of experimental data from controlled human exposure, animal, and in vitro studies is required to provide a sufficient weight of evidence to support regulatory policy.
Indoor Air Pollution
Indoor air quality has been increasingly recognized as an important determinant of human health. As levels of outdoor ambient air pollution have decreased throughout the developed world, and because residents of these countries spend the majority of their time indoors, the relative impact of indoor air pollution has increased. To characterize personal exposure to air pollution, indoor air quality must be taken into account ( Table 74-4 ).
Category of Pollutant | Indoor Pollution Source |
---|---|
Combustion (e.g., NO 2 , CO, PM 2.5 , PAHs) | Secondhand smoke |
Gas stoves, ovens, heaters, and fireplaces | |
Wood smoke (stoves, fireplaces) | |
Kerosene heaters | |
Candles and incense | |
Building materials (e.g., formaldehyde, volatile organic compounds) | Plywood |
Particle board | |
Carpeting | |
Paints | |
Cleaning materials (e.g., ammonia gas, chlorine gas, choramine) | Bleach, ammonia, detergents |
Sources of Indoor Pollution
The concentrations of indoor air pollutants depend on both indoor and outdoor emission sources ( Fig. 74-1 ). Outdoor emission sources determine outdoor pollution concentrations, which, in turn, affect indoor air quality. The degree of penetration of outdoor pollution into the indoor environment depends largely on the rate of air infiltration from outdoors to indoors. The rate of air infiltration is a complex function of the “tightness” of building construction and insulation, building location and orientation, number of exterior walls and windows, surrounding terrain and barriers, wind speed and direction, indoor-outdoor temperature gradient, and ventilation system type (e.g., heating, ventilation, air-conditioning system) and efficiency.

Time-activity patterns will influence personal exposure. The relative time spent indoors versus outdoors will affect the contribution of indoor pollutants to total personal exposure. Time spent in specific locations within the home will affect exposure to particular pollutants, as the distribution of indoor pollutant concentrations will vary among different zones of a building, especially for pollutants generated indoors. In addition, activity level will affect metabolic rate and ventilatory rate, which will also influence personal exposure to indoor pollutants.
Indoor Combustion: Major Source of Indoor Air Pollution
The major source of indoor pollution is from combustion, particularly secondhand smoke (SHS) exposure, gas stove use, and wood burning in stoves and fireplaces. Kerosene space heaters are also an important source of indoor air pollution. Table 74-4 shows the major indoor pollutants that are released from these sources and are associated with adverse pulmonary effects.
Secondhand Smoke and Obstructive Lung Disease
SHS is perhaps the best known and studied indoor pollutant. Strong evidence implicates it as a cause of lung cancer, coronary heart disease, and decreased life span. In addition to these adverse health effects, SHS contains respiratory irritants, such as SO 2 , ammonia, acrolein, and formaldehyde. SHS is a complex mixture containing thousands of gas-phase and particulate substances. Homes where smoking is allowed have PM 2.5 levels up to 10 times higher than nonsmoking homes. Workplace exposures can be even higher, especially in bars and casinos.
Passive smoking has also been linked with allergic phenomena, such as elevated serum immunoglobulin E levels. As a consequence, SHS has the potential to induce new cases of asthma through irritant or sensitizing mechanisms. The results of multiple studies provide evidence that SHS exposure is causally associated with new-onset asthma among children.
Exposure to SHS also appears to be associated with new-onset asthma in adulthood. Cross-sectional, case-control, and cohort studies have suggested a link between SHS exposure and adult-onset asthma. SHS exposure in the workplace, in particular, appears to be related to adult asthma induction.
Beyond asthma induction, SHS exposure is a likely cause of asthma exacerbation in both children and adults. Parental smoking causes increased symptoms, poorer pulmonary function, and other indicators of worsened asthma control among children with established asthma. Similarly, SHS exposure, both at home and at work, appears to cause asthma exacerbation among adults. Moreover, the negative impact of SHS exposure on pulmonary function appears to be greater among adults with asthma than in the general population.
Exposure to SHS may also cause respiratory symptoms and impaired lung function among nonasthmatic individuals. In a cohort of older adults, lifetime home and workplace exposure to SHS was associated with a more rapid decline of lung function during a decade of follow-up. Studies of bartenders who had high occupational exposures to SHS have revealed a strong link between SHS and poorer respiratory health. Since smoke-free workplace laws have been implemented in several different locations around the world, bartenders and restaurant workers have experienced a reduction of respiratory symptoms and improved lung function.
Emerging evidence now suggests that SHS exposure may also be a cause of COPD independent of personal cigarette smoking. A population-based study from the United States found that both cumulative home and work SHS exposure was associated with a greater risk of COPD, controlling for potential confounders. A recent study from China found that self-reported cumulative lifetime SHS exposure at home and work was related to a greater risk of COPD. Other epidemiologic studies also support an association between SHS exposure and the development of COPD. Among patients with established COPD, SHS exposure may increase the risk of respiratory symptoms, poor health status, and disease exacerbations.
Gas Stove Exposure: Cause of Asthma Exacerbation?
Cross-sectional epidemiologic studies demonstrate an increased risk of childhood asthma in homes with gas stoves, which are a major source of indoor NO 2 , compared with homes with electric stoves, although a recent birth cohort study did not. In adults with established asthma, a prospective panel study found an association between gas stove use and increased risk of respiratory symptoms, restricted activity, and emergency department visits. Another time-series analysis found a negative impact of gas stove use on daily peak expiratory flow and respiratory symptoms. In contrast, a longitudinal U.K. cohort study found no effect of gas stove exposure on persistence of adult asthma or on respiratory symptoms among asthmatics ; the European Community Respiratory Health Survey investigators found no clear link between gas stoves and asthma symptoms. Moreover, a prospective cohort study of adults with asthma found no impact of gas stove exposure on asthma outcomes and, in a population-based sample of U.S. adults, no association between gas stove exposure and pulmonary function impairment was observed. Overall, the evidence has not been sufficient to implicate gas stove use as an exacerbating factor in preexisting adult asthma. Among children with asthma, however, there is stronger evidence of a deleterious effect of indoor NO 2 exposure and poorer asthma control.
Wood Smoke Exposure—Respiratory Health Effects
Wood smoke, which is produced from domestic fireplace or wood stove use, contains potent respiratory irritants such as formaldehyde, acrolein, nitrogen oxides, and SO 2 . It is also a major source of particulate air pollution. Exposure to extremely high wood smoke levels has been linked with respiratory problems. After a work shift, forest firefighters experience an acute decrement in pulmonary function. Similarly, wildland fires have been associated with increased respiratory symptoms and health care utilization for respiratory problems. In developing countries, wood smoke from domestic cooking and heating in poorly ventilated homes has been associated with chronic bronchitis and COPD. The exposure levels in such settings are extremely high and are likely the major cause of COPD in women in the developing world, who tend not to smoke cigarettes. Cooking with other biomass fuels, such as crop residues or dung, and with coal can also lead to chronic respiratory symptoms and airflow obstruction. Cooking with wood and/or charcoal has been associated with risk of COPD in developed countries as well.
The respiratory health effects of residential biomass smoke exposure on asthma are not as clear. Previous studies have reported conflicting results. However, an analysis from the International Study of Asthma and Allergy in Children, which included more than 250,000 children from over 31 countries, did find an association between use of an open fire for cooking and prevalence of asthma symptoms and reported asthma. Exposures to wood smoke in developed countries are usually at much lower levels than in countries where a large proportion of the population cooks on open fires, so it is less clear how such exposures contribute to asthma burden.
Respiratory Effects of Kerosene Heater Use
Kerosene heaters can substantially increase indoor levels of fine particles (PM 2.5 ), sulfate aerosol (SO 4 2− ), and acidic aerosol (H + ), as well as CO. Kerosene is similar to diesel fuel in chemical composition. The existing evidence, however, is inconclusive about whether kerosene heater use is a cause of respiratory and asthma symptoms in developed countries. In developing countries where kerosene may also be used for lighting using inefficient wick lamps, this fuel has been associated with increased risk of respiratory infections, especially tuberculosis.
Other Indoor Pollutants—“Toxic” Indoor Environment
Besides indoor combustion, there are many other sources of indoor pollutants. Building materials, such as plywood or particle board, and carpeting can emit formaldehyde; furniture may release formaldehyde and volatile organic compounds (VOCs); paints, cleaning compounds, and photocopiers may release VOCs; and radon can arise from soil sources. Cleaning with bleach and other compounds can release chlorine and ammonia gas, which are airway irritants. Combination of bleach and ammonia or other nitrogen sources can also produce chloramine, which may induce upper and lower respiratory tract symptoms. Of these substances, radon exposure has been associated with lung cancer. Exposure to chlorine, chloramine, and VOCs may be linked with respiratory symptoms, some of which suggest asthma, but further evidence is necessary before final conclusions can be drawn.
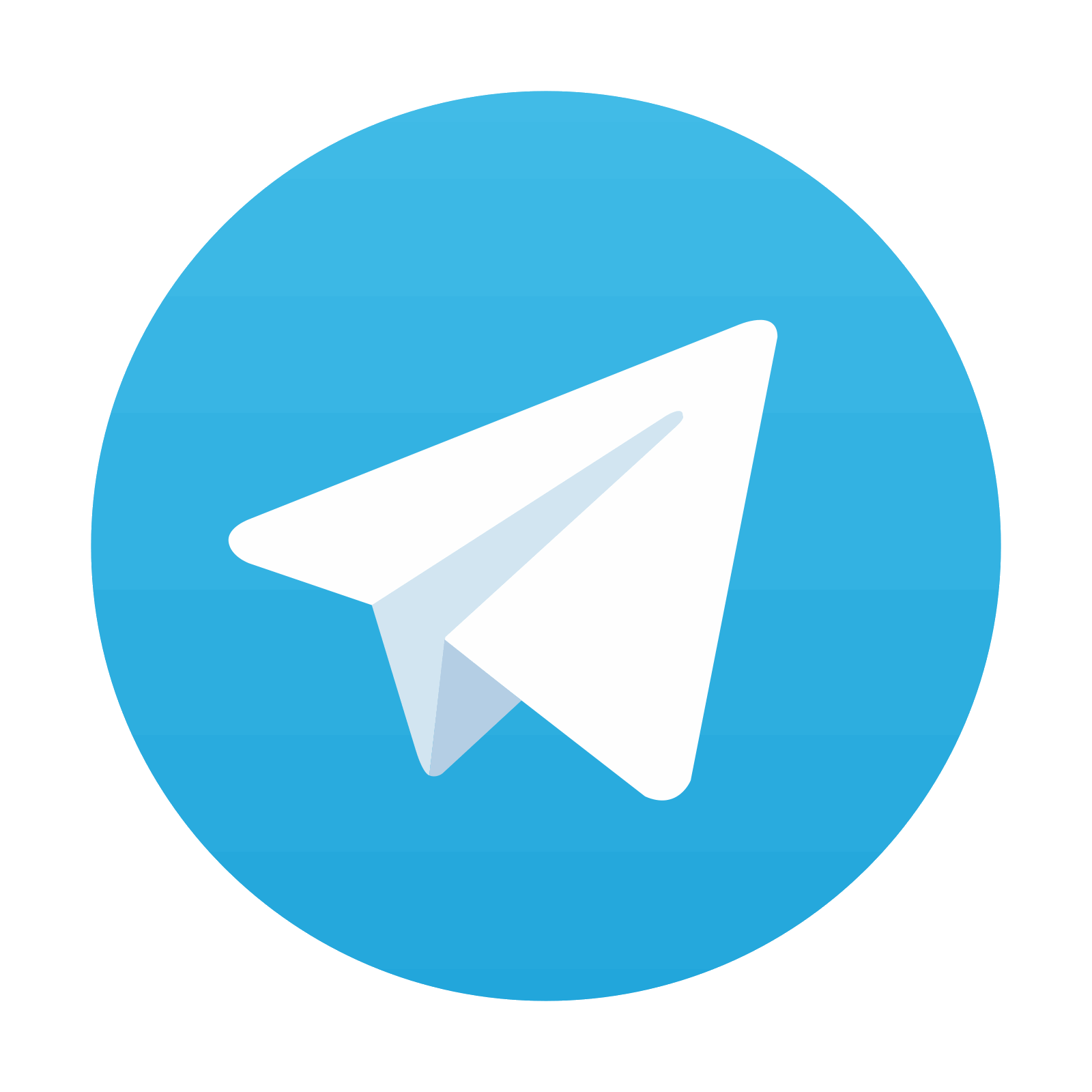
Stay updated, free articles. Join our Telegram channel
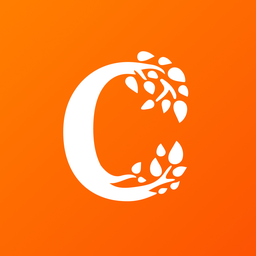
Full access? Get Clinical Tree
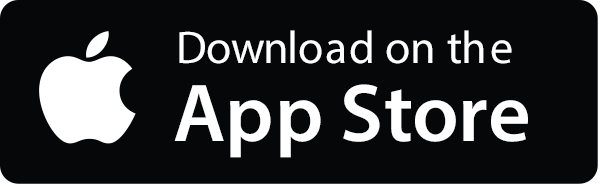
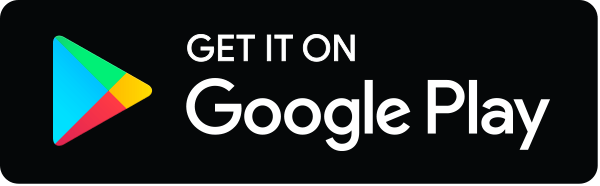