Chun Soo Park1 and James S. Tweddell2 1Asan Medical Center, University of Ulsan College of Medicine, Seoul, South Korea 2Cincinnati Children’s Hospital Medical Center and University of Cincinnati, Cincinnati, OH, USA Hypoplastic left heart syndrome (HLHS) is a group of congenital cardiac malformations consisting of various degrees of underdevelopment of the left heart and the aorta, resulting in significant obstruction to blood flow and the inability of the left heart to support the systemic circulation [1, 2]. The concept of this complex anomaly was first described by Lev in 1952 as a hypoplasia of the aortic tract complexes [3], and the term HLHS, which is widely accepted to describe this entity, was introduced by Noonan and Nadas in 1958 [4]. HLHS is a rare disease constituting 1.4–3.8% of congenital heart disease (CHD) in autopsy series, with an incidence of 0.016–0.036% of live births [5]. Despite this low incidence, HLHS comprises 23% of cardiac deaths during the first week of life and 15% of cardiac deaths within the first month of life [6–10]. Males are known to be affected more frequently than females [6, 11]. There are several converging lines of evidence supporting a genetic cause of HLHS. The recurrence risk in families with one affected child is 0.5–2% [10, 12], and recurrence rate of any CHD in first‐degree relatives of patients with HLHS ranges from 12% to 19 % [13–15]. These are predominantly left‐sided obstructive lesions, including aortic stenosis, bicuspid aortic valve, and arch obstruction. About 30% of HLHS cases are associated with defined chromosomal defects, genetic syndromes, or extracardiac congenital anomalies [16]. Heritable syndromes including Noonan [17], PAGOD [18, 19], Kabuki [20], Holt–Oram [21], CHARGE [16], and Turner [22, 23], along with trisomies 13, 18, and 21 [16], have been reported with HLHS. Environmental triggering factors have also been implicated in the development of HLHS. The Baltimore‐Washington Infant Study and eastern Wisconsin study demonstrated that the occurrence of HLHS was concentrated in a specific region, which was characterized by industrial land use [24, 25] and release of toxic chemicals [25]. Both studies suggest the crucial role of environmental exposure in the development of HLHS. Several studies also demonstrated a seasonal variation in the occurrence of HLHS, which suggests a significant role of infection in the development of HLHS [26, 27]. With the introduction of fetal echocardiography, it has become evident that congenital heart diseases including HLHS evolve throughout gestation. There have been a number of studies suggesting that diminished inflow or obstruction to the outflow of the left ventricle might cause the in utero development or progression of HLHS [28–35]. In one animal study using chick embryos, left ventricular hypoplasia could be replicated with occlusion of the mitral valve with suture [36]. This seems unlikely to lead to the development of HLHS in humans, because mitral stenosis occurring as a primary lesion results in enlargement of the left atrium, rather than hypoplasia [11]. It is likely that the left ventricular outflow tract lesion occurs first and is the inciting lesion [37], as the degree of outflow obstruction is uniformly of the greatest severity in HLHS among the left‐sided obstructive lesions. In the presence of aortic stenosis or atresia, one mechanism for the exacerbation and acceleration of the development of HLHS is related to the altered compliance of the left ventricle. This can be secondary either to the development of left ventricular hypertrophy or to the development of left ventricular dilatation and dysfunction. Increasing left atrial pressure with disease progression leads to decreasing flow across the foramen ovale and even its premature closure, resulting in the loss of impetus for left ventricular growth due to preload starvation. Some investigators [34, 38] have proposed the following prenatal indicators for the development of HLHS: a smaller mitral valve and ascending aorta, a decreased rate of growth for all left heart structures, reversal of flow across the foramen ovale, retrograde transverse aortic arch flow, and a monophasic mitral inflow. For the fetus with aortic stenosis, serial echocardiographic examination needs to be performed, focusing on the growth of left heart structures and the patterns of blood flow across the foramen ovale and transverse aortic arch. The main objectives of fetal echocardiography for HLHS are to follow the evolution of HLHS and to diagnose restrictive or intact atrial septum prior to delivery, since these patients may benefit from prenatal intervention or planned delivery [39–42]. The HLHS encompasses a spectrum of cardiac malformations that are characterized by significant underdevelopment of the components of the left heart and the aorta, including the left ventricular cavity and mass (Figure 32.1) [1]. Aortic atresia with mitral atresia or stenosis is the most common abnormality, accounting for more than half of the patients with HLHS [43]. The ascending aorta and aortic root are markedly hypoplastic, with the ascending aorta serving only as a conduit for coronary arterial supply. In patients with aortic stenosis, the aortic valve leaflets are frequently dysplastic, and rarely may be even absent [44, 45]. At the milder end of the spectrum, some patients with aortic stenosis and mitral stenosis can overlap with patients with critical aortic stenosis that might be amenable to biventricular circulation. The ascending aorta shows varying degrees of hypoplasia. In a large single‐center experience, the mean ascending aortic diameter was 3.3 ± 1.7 mm and 40–55% of patients had an ascending aorta of <2 mm [46, 47]. The components of the arch itself are also variably hypoplastic, and a localized coarctation of the aorta is commonly present in more than 75% of patients (Figure 32.2) [48, 49]. Ductal tissue can encircle the aortic lumen and extend from the ductal orifice proximally and distally [50], which has important surgical implications for selecting the strategy of arch repair. The mitral valve is almost always stenotic or atretic. Aortic atresia with mitral atresia is the most common anatomic subtype and occurs in 36–46% of patients undergoing stage 1 palliation, followed by aortic stenosis with mitral stenosis in 23–26%, and aortic atresia with mitral stenosis in 20–29% [46, 51, 52]. A recent pathologic review of 119 specimens mostly entered into an archive prior to surgical intervention had slightly different anatomic subgroups. The phenotypes included mitral and aortic atresia (30%), mitral and aortic stenosis (22%), and mitral stenosis with aortic atresia (48%). In this series the variability in the morphologic findings supported the notion that the lesions seen represent acquired disease occurring subsequent to closure of the embryonic interventricular communication, rather than representing abnormal embryogenesis [28]. Endocardial fibroelastosis of the left ventricle is almost always present in association with aortic atresia and open mitral valve, while it is never present in association with mitral atresia [28, 53]. Endocardial fibroelastosis is thought to develop as a result of subendocardial ischemia caused by high left ventricular intracavitary pressure throughout the cardiac cycle [53]. Coronary artery abnormalities including ventriculocoronary connections and thickening of the intima are more common in the subgroup of patients with aortic atresia and mitral stenosis [11, 54–57]. Abnormalities of the tricuspid valve are found in 35% of the HLHS patients and in up to 50% of those with mitral stenosis [58, 59]. Hypertrophy of the left ventricle, as occurs with severe outflow tract obstruction with a patent mitral valve, can result in deformation of the interventricular septum, leading to distortion of the subvalvar apparatus of the tricuspid valve and resulting in regurgitation [53]. Tricuspid valve abnormalities can also be acquired as annular dilatation secondary to volume overload of the single right ventricle, or chordal elongation with leaflet prolapse caused by subendocardial ischemia at the time of presentation or following the Norwood procedure [60]. Since the aforementioned features, such as endocardial fibroelastosis coronary artery abnormalities and the potential for tricuspid valve insufficiency, are more prevalent in patients with aortic atresia and mitral stenosis, this subgroup has been considered to be at increased risk for mortality after the Norwood procedure [54, 57, 61]. Most patients with HLHS have an intact ventricular septum. If there is a ventricular septal defect, it is usually small [1]. The atrial septum will be intact or highly restrictive in up to 10% of patients with HLHS [11]. Premature closure or restriction of the patent foramen ovale, which has been postulated as a cause of HLHS, can occur as a primary event or as a secondary event to left ventricular outflow obstruction [60, 62]. An intact atrial septum can lead to an increase in left atrial pressure and left atrial hypertrophy, along with arterialization of the pulmonary veins and lymphangiectasia. Even if appropriate and timely postnatal intervention is performed, these changes in utero can persist and result in postnatal demise [11, 60]. The atrial septum can be deviated to the left on its superior aspect and attached to the roof of the left atrium, most often in those with aortic and mitral atresia [1, 63]. Nonexistence of atrial septum in the expected location can be mistaken for a large atrial septal defect [1]. Figure 32.1 (A) A four‐chamber preparation that demonstrates the small, slit‐like left ventricle that is associated with the variant of hypoplastic left heart syndrome that has mitral atresia and aortic atresia. In this case the mitral valve is imperforate and the aortic valve is not seen. Secondary to the mitral atresia, blood is not able to enter the left ventricle and it maintains a thin muscular wall without endocardial fibroelastosis. (B) A hypoplastic left ventricle that has a globular appearance with extensive muscular walls and endocardial fibroelastosis, which is typical when there is mitral stenosis and aortic atresia. (C) The variant with mitral and aortic stenosis. The hypoplastic left ventricle resembles that seen in (B) with extensive endocardial fibroelastosis. (D) A long‐axis view and what is sometimes referred to as “hypoplastic left heart complex.” With this variant the small size of the left ventricle, the mitral valve, and aortic valve are all comparable to one another. Note the focal endocardial fibrosis along the septal surface, with the remainder of the endocardium spared. Venous anomalies are uncommon in association with HLHS. Anomalous pulmonary venous connection or drainage occurs in 6% of patients [64]. Some of the patients with a highly restrictive or intact atrial septum will have a levoatriocardinal vein present that allows for decompression of the pulmonary venous return [65]. Persistent left superior caval vein occurs in about 15% of HLHS patients [60]. Apart from ventriculocoronary connections, other forms of coronary abnormalities are uncommon. Anomalous origin of coronary arteries has been reported anecdotally [66–70]. Even though the coronary arteries originate from a small ascending aorta, the diameters of the coronary arteries and ostia are usually normal [71]. A hypoplastic left ventricle that is not capable of supporting the systemic circulation can be seen in patients with interrupted aortic arch and critical left ventricular outflow obstruction with a large ventricular septal defect, unbalanced atrioventricular septal defect, or double‐outlet right ventricle [60]. These combinations can be categorized as a variant of HLHS and the treatment options are the same as for HLHS. In rare instances with the presence of a large ventricular septal defect, the development of the left ventricular chamber and mitral valve may be near normal size and allow for a complex biventricular repair. Figure 32.2 (A) An anterior view of the arterial trunks in a heart that has mitral and aortic valvar atresia. This yields a very tiny aortic root and ascending aorta, which basically serves as a conduit to supply the coronary arteries with oxygenated blood in retrograde fashion. Note the large pulmonary trunk and patent arterial duct. (B) The left atrioventricular junction with a hypoplastic left ventricle and stenotic mitral valve, along with a restrictive atrial septum or premature closure of the oval foramen. The left aspect of the flap valve is marked with yellow dots, with thickening of the left atrial endocardium and muscular wall. (C) An explanted heart with a wedge‐like section removed from the hypoplastic left ventricle to demonstrate the communication between an ectatic, epicardial coronary artery and the left ventricular cavity. (D) The ascending aorta and aortic arch opened in this anterior view of the arterial trunks. The ascending aorta is tiny and there is a raised, preductal ridge or coarctation (red arrow) that is also associated with the opening of the left subclavian artery. Increasingly the diagnosis of HLHS is made by fetal echocardiogram prior to delivery. This allows for parental counseling and delivery planning. After delivery, prostaglandins should be administered to keep the arterial duct open, and echocardiogram needs to be performed to confirm the diagnosis. While most neonates with HLHS can be initially managed medically after delivery, the presence of a restrictive atrial septal defect results in left atrial hypertension, leading to pulmonary congestion. This requires emergent intervention with transcatheter septostomy or septectomy. The survival benefit of prenatal diagnosis on surgical outcomes for HLHS is inconsistent [72–74]. It has been shown that greater distance from the receiving cardiac surgical center, obstructed pulmonary venous return, or a major extracardiac congenital abnormality is associated with increased preoperative death [75, 76]. There is some evidence that prenatal diagnosis may be protective against brain white‐matter injury [77]. If the infant has not been diagnosed in fetal life, the timing and severity of presentation vary depending on ductal patency and the degree of atrial‐level restriction. Some neonates with HLHS and widely patent atrial septal defect may initially look normal and have a more delayed presentation. Since ductal closure is inevitable and pulmonary vascular resistance continues to decrease after birth, the combination of systemic outflow obstruction and pulmonary overcirculation will cause eventual deterioration, with poor feeding, respiratory distress, and progression to congestive heart failure and shock. Peripheral pulses are palpable early, but are reduced later as ductal closure ensues. Hepatomegaly is common and is generally seen in infants with a delayed presentation. On cardiac examination, the second heart sound is single and accentuated. A mid‐systolic ejection murmur may be heard along the left sternal border. The chest radiograph is frequently nondiagnostic, but can be helpful to guess the degree of atrial‐level restriction. In the infant with a highly restrictive atrial septal defect, it shows relatively normal heart size and pulmonary edema. Cardiomegaly and pulmonary plethora are seen in infants with a nonrestrictive atrial septal defect. The electrocardiogram shows right‐axis deviation and right ventricular hypertrophy, but may not be distinctly different from the normal electrocardiogram of the neonate. The diagnosis of HLHS can be readily made by two‐dimensional echocardiography with color Doppler and is usually sufficient to make a surgical plan (Figure 32.3). The cardiac anatomy and physiology should be investigated, focusing on the size of the ascending aorta, branching patterns of the arch vessels, the degree of restriction of the atrial septum, the function of the right ventricle, the morphology and function of the tricuspid and pulmonary valves, the pulmonary vein anatomy, patency and flow characteristics of the ductus, and the coronary arterial system with the presence of ventriculocoronary connection [11]. Cardiac catheterization is used as an adjunct tool to better identify pulmonary venous anomalies, or coronary anomalies. Also, in the setting of a severely restrictive atrial septum, catheter intervention or emergent surgical atrial septectomy may be lifesaving [60]. Figure 32.3 Two‐dimensional echocardiography with multiple imaging views from a patient with hypoplastic left heart syndrome (aortic atresia with mitral atresia). (A) Apical four‐chamber view. The left heart structures are much smaller than the right heart structures. The right ventricle is forming the cardiac apex. (B) Parasternal short‐axis view. Blood from the heart coming out through the main pulmonary artery is passing through the unobstructed patent arterial duct (arrow). (C) Subcostal view (coronal). The atrial septum is seen (arrows), and color Doppler imaging identifies the unobstructed interatrial communication. (D) Suprasternal notch view (long axis). The ascending aorta is markedly hypoplastic (arrows), and color Doppler imaging demonstrates retrograde flow through the aortic arch. LA, left atrium; LV, left ventricle; MPA, main pulmonary artery; RA, right atrium; RV, right ventricle. After birth, the medial smooth muscle fibers in the arterial duct contract, which results in wall thickening, lumen obliteration, and shortening of the duct. Functional complete closure usually occurs within 24–48 hours of birth in term neonates [78]. For this reason, prostaglandin E1 (PGE1) should be administered immediately to sustain ductal patency in newborns with diagnosed or suspected HLHS. Once ductal patency is confirmed by echocardiogram, the PGE1 infusion rate can be adjusted (as low as possible) to avoid the known side effects of hypotension and respiratory depression [79, 80]. Patients with impaired ventricular function or shock will benefit from inotropic support. A decision on the use of inotropes is made by clinical features and echocardiographic findings. Since dose escalation of catecholamines may result in an undesired increase in systemic vascular resistance (SVR) that subsequently raises the pulmonary to systemic flow ratio (Qp : Qs), inodilator therapy with milrinone (a phosphodiesterase inhibitor) can be used for patients with elevated Qp : Qs and systemic hypoperfusion [60]. For the stressed neonate with increased SVR, low‐dose sedation can be used to reduce agitation and work of breathing and improve systemic oxygen balance without mechanical ventilation. Most patients with HLHS become physiologically vulnerable due to the unrestricted pulmonary blood flow, as pulmonary vascular resistance (PVR) falls after birth. In order to avoid or minimize pulmonary overcirculation, PVR can be increased with manipulation of alveolar gas tension, frequently assisted by controlled mechanical ventilation. The use of positive end‐expiratory pressure and avoidance of hyperventilation can be helpful. In addition, the use of inspired carbon dioxide (CO2) can be effective in attenuating the pulmonary blood flow by increasing PVR [81–83] and improving systemic oxygen delivery [84]. Although excessive supplemental oxygen, a potential pulmonary vasodilator, should generally be avoided, for preoperative patients with parenchymal lung disease the use of supplemental oxygen to prevent pulmonary vein desaturation is essential. Any of the aforementioned preoperative management strategies should be used with sufficient monitoring, as they can pose potential risks to the neonate awaiting surgery for HLHS. For the patient in whom preoperative stabilization is difficult, early surgical intervention should be considered [85, 86]. For the patient with shock and anasarca or contraindications to cardiopulmonary bypass, bilateral branch pulmonary artery banding should be considered [87, 88]. For the patient with HLHS, like other functional single‐ventricle lesions, a three‐step staged surgical strategy is now widely adopted. The universal aim of the neonatal stage is to create a stable parallel circulation that will permit somatic growth and lung maturation to support progression to a bidirectional cavopulmonary connection and eventual completion Fontan (or total cavopulmonary connection). For the patient with HLHS, the first stage requires relief of systemic outflow obstruction, including provision of coronary artery blood flow, an appropriately restricted amount of pulmonary blood flow, and creation of a nonrestrictive atrial septal communication. Current strategies consist of the Norwood procedure with either a Blalock–Taussig–Thomas (BTT) shunt or a right ventricle to pulmonary artery conduit (Sano modification) or hybrid palliation (Figure 32.4) [89, 90]. Usually, a superior cavopulmonary anastomosis is established as stage 2 palliation, to achieve a more efficient circulation, improve oxygenation, and unload the single ventricle [91]. Finally, the staged pathway culminates in a Fontan procedure, where the inferior caval vein (IVC) is routed to the pulmonary arteries with or without fenestration [89, 92, 93]. The goals of neonatal palliation for HLHS include relief of systemic outflow obstruction, provision of unobstructed coronary blood flow, creation of a nonrestrictive atrial septal communication to prevent pulmonary venous hypertension, and establishing reliable and appropriately limited source of pulmonary blood flow. Since Norwood and colleagues reported the first successful palliation of HLHS in 1980 [89, 94], the surgical approach has changed over time and continues to evolve. A successful Norwood palliation requires optimal surgical technique and cardiopulmonary bypass strategies to permit safe and precise reconstruction. Preoperative stabilization is essential for optimal surgical outcome. Except in the rare patient unresponsive to PGE1 or with a highly restrictive atrial septal defect, surgery should not take place until the circulation has been stabilized and low cardiac output or acidosis has been resolved. Surgery is typically scheduled electively about 2–5 days after presentation. Figure 32.4 The three options for stage 1 palliation of hypoplastic left heart syndrome. (A) A Norwood procedure with a Blalock–Taussig–Thomas shunt. (B) A Norwood procedure with a right ventricle to pulmonary artery conduit (Sano procedure). (C) The hybrid procedure, which includes bilateral pulmonary artery banding, maintenance of ductal patency with a stent, and creation of an atrial septal defect. Source: [60] / Wolters Kluwer Health. After a median sternotomy, the pericardium is opened and the vascular structures are mobilized. The innominate vein is mobilized and looped with a tape to allow for atraumatic retraction and facilitate exposure of the brachiocephalic vessels. For conducting cardiopulmonary bypass, a single venous cannulation is commonly used and arterial cannulation can be established directly through the main pulmonary artery [95] or innominate artery, either directly or via a synthetic graft (Figure 32.5) [96–98]. Cardiopulmonary bypass is instituted and the patient is cooled to a bladder temperature of 18–20 °C over 30 minutes using pH‐stat perfusion strategy. With initiation of cardiopulmonary bypass, the branch pulmonary arteries are snared and a ventricular vent is placed through the right atrial free wall and directed across the tricuspid valve into the ventricle to prevent ventricular distension. The main pulmonary artery is divided just proximal to the bifurcation of the branch pulmonary arteries, and the distal pulmonary artery confluence is closed directly or with a patch. In this step, care must be taken to avoid air embolism in patients with a patent aortic valve. The arterial duct is ligated and the proximal descending aorta is mobilized including division of the first two or three sets of intercostal arteries (Figure 32.6). Circulatory arrest is established at a bladder temperature between 18 and 20 °C, cardioplegia is delivered through the arterial cannula, and atrial septectomy is accomplished. Figure 32.5 Cardiopulmonary bypass. Arterial cannulation is established through the innominate artery (Innom. a.) via a Gore‐Tex tube graft and venous cannulation through the right atrial appendage. A ventricular vent is inserted through a right atrial free wall to prevent ventricular distension. The brachiocephalic vessels and the ductus are snared. Ao, aorta; PA, pulmonary artery; PDA, patent arterial duct; RA, right atrium. Source: [101], Tweddell JS, et.al., (2012). Reproduced with permission from Elsevier. After completing the atrial septectomy and complete mobilization of the ascending aorta, arch, brachiocephalic vessels, and proximal descending thoracic aorta, cerebral perfusion is initiated through the innominate artery in order to minimize the period of deep hypothermic circulatory arrest. The flow rate for antegrade cerebral perfusion is 40–60 mL/kg/min and is guided by right radial arterial line pressure and/or near‐infrared spectroscopy (NIRS). The aortic isthmus and the ductus arteriosus are divided, and all ductal tissue is excised from the descending thoracic aorta. An interdigitating arch reconstruction is performed in order to reduce the rate of recurrent arch obstruction [99–101]. The incision of the aortic arch begins at the aortic isthmus, extends proximally down the ascending aorta, and ends just above the adjacent “kissing” point between the ascending aorta and the pulmonary root. Great care must be taken with the diminutive ascending aorta to avoid spiraling this incision. A cutback is made in the pulmonary root at the site of implantation of the ascending aorta, extending about 2 mm below the sinotubular junction just left of the commissure that faces the ascending aorta. It is also important to end the aortic incision 2–3 mm cephalad to the level of the pulmonary root cutback. This results in a small degree of redundancy in the proximal ascending aorta and ensures a nonstenotic connection between the aortic root and the pulmonary root (Figure 32.7). A cutback is also made into the posterior left lateral descending thoracic aorta. The descending thoracic aorta has been adequately mobilized to allow it to be advanced to the arch and distal ascending aorta. The distal arch is interdigitated into the cutback in the proximal descending aorta and the posterior walls sutured together with 7‐0 polypropylene suture. A second small cutback is made in the anterior portion of the descending aorta. The connection between the adjacent sites on the ascending aorta and the cutback in the pulmonary root are accomplished with 7‐0 or 8‐0 polypropylene in the case of a very small (<2 mm) ascending aorta (Figure 32.8A). A patch is used for completion of the arch reconstruction and creation of the neo‐ascending aorta. This patch can be pulmonary homograft, pericardium, or porcine small intestinal submucosa (CorMatrix, Alpharetta, GA, USA), depending on the surgeon’s experience or preferences (Figure 32.8B). When the junction of the ascending aorta and pulmonary root is reached, the excess patch is trimmed and the proximal connection between the patch and the pulmonary root is completed (Figure 32.8C). In the case of establishing the pulmonary blood flow with a right ventricle (RV) and pulmonary artery (PA) conduit, the site for the proximal connection should be identified while the pulmonary root is open, and should be at least 1 cm below the nadir of the pulmonary valve cusp, and away from any large epicardial coronary artery [101]. Figure 32.6 The main pulmonary artery is divided just proximal to the bifurcation of the branch pulmonary arteries and the distal stump is closed with a patch. The arterial duct is ligated and the proximal descending aorta is mobilized during cooling. An insulated electrocautery is used at low settings to divide the first two or three sets of intercostal arteries. Source: [101], Tweddell JS, et.al., (2012). Reproduced with permission from Elsevier. Once arch reconstruction is completed, the source of pulmonary blood flow should be established. Until Sano and coworkers reintroduced the RV–PA conduit modification of the Norwood procedure in 2003 [102], pulmonary blood flow had been provided by a modified BTT shunt. A theoretical advantage of the RV–PA conduit is the elimination of diastolic runoff, and increased diastolic pressure with improved coronary perfusion pressure [103–106]. Potential disadvantages include a negative effect on RV function and ventricular arrhythmias due to the ventriculotomy, impaired growth of the PAs, and the need for an earlier stage II procedure [107–109]. The Single Ventricle Reconstruction trial, a multicenter, randomized controlled trial of 549 patients comparing the RV–PA conduit with the modified BTT shunt, showed that transplant‐free survival at 12 months was better with the RV–PA conduit, but the RV–PA conduit group had more unintended interventions [108]. In longer follow‐up of this cohort, the RV–PA conduit was no longer associated with superior transplantation‐free survival at 3 years [110], presumably related to the deleterious effects of the ventriculotomy. In contrast, a prospective observational study using propensity matching conducted by the Congenital Heart Surgeons’ Society (CHSS) showed a sustained survival benefit associated with the RV–PA conduit group [111]. This discrepancy can be potentially explained by the enrollment of more recent patients in the CHSS study with more experience with the RV–PA conduit [112, 113]. Figure 32.7 After delivering cardioplegia and completing the excision of the atrial septum, regional cerebral perfusion is initiated. The aortic isthmus is divided and all ductal tissue is excised. The undersurface of the aortic arch is incised, beginning at the aortic isthmus and extending proximally down the ascending aorta. A cutback is made in the pulmonary root at the site of implantation of the ascending aorta. It is also important to end the aortic incision 2–3 mm cephalad to the level of the pulmonary root cutback. A cutback is made into the posterior left lateral descending thoracic aorta. Source: [101], Tweddell JS, et.al., (2012). Reproduced with permission from Elsevier. When the RV–PA conduit is selected, it can be performed under full flow during the rewarming phase. In order to prevent proximal obstruction of the RV–PA conduit, a dunk technique is performed by inserting a ringed Gore‐Tex (W.L. Gore, Newark, DE, USA) tube graft through the right ventricular free wall (Figure 32.9A,B) [101, 114]. The conduit can be positioned to the right or left of the neo‐ascending aorta, but the right side is preferred as it makes the stage 2 procedure easier. The diameter of the conduit is determined, depending on the patient’s weight and the course of the conduit, usually 5 mm if the conduit is brought to the left or 6 mm if the conduit is brought to the right. The difference in diameter is due to the slightly longer right‐sided conduit. If the modified BTT shunt is elected as a source of pulmonary blood flow, the arterial cannula is transferred from the Gore‐Tex tube graft to the neo‐ascending aorta first [100]. Distal anastomosis can be accomplished during the rewarming phase of cardiopulmonary bypass. A more centrally positioned shunt helps to promote equivalent growth of the branch pulmonary arteries (Figure 32.9C). Modified ultrafiltration is conducted routinely following successful separation from cardiopulmonary bypass. Hybrid approaches, branch PA banding with or without ductal stenting, followed by either a conventional Norwood procedure or a Norwood procedure combined with a bidirectional Glenn shunt (comprehensive stage 2), has been reported as a rescue intervention in high‐risk neonates [87, 88, 115] or as a routine strategy [116, 117] to avoid the risk of cardiopulmonary bypass [116–120]. Important shortcomings of hybrid palliation include creation of an adequate atrial septal defect and the risk of retrograde arch obstruction, especially for the patient with aortic atresia, which can result in cerebral and coronary ischemia [121, 122]. The outcomes from two leading centers using hybrid palliation as a routine stage 1 strategy are promising and suggest that a commitment to this approach can yield excellent results, albeit at the expense of increased interstage interventional catheter‐based procedures [118, 119]. After the Norwood procedure, the output of the postischemic single RV is limited. This physiologic vulnerability peaks in the first 6–12 hours postoperatively [123]. To ensure adequate oxygen delivery to the tissues, the limited output from the single RV must be partitioned precisely between the systemic and pulmonary circulations. Assessment of cardiac output, pulmonary to systemic flow ratio, circulatory wellbeing by physical examination, and routine perioperative monitoring are notoriously inaccurate; therefore objective assessment tools are necessary [124]. Mixed venous saturation (SvO2) can be measured to determine if oxygen delivery is adequate and provides direct information concerning the pulmonary to systemic flow ratio (Figure 32.10). NIRS takes advantage of the difference in absorption of light by oxygenated and deoxygenated hemoglobin and can determine the relative oxygen content of the tissue beneath the probe. Both SvO2 and NIRS data have been used as primary markers for systemic oxygen delivery targeting SvO2 >50%, cerebral rSO2 >50%, and somatic rSO2 >60%, to minimize organ dysfunction and the risk of secondary multisystem organ dysfunction [74, 125, 126]. Management based on SvO2 or NIRS as a surrogate is shown in Table 32.1. Based on a large perioperative database, it appears that two‐site NIRS monitoring combined with heart rate and blood pressure is an appropriate target for goal‐directed therapy, with the added benefit of being noninvasive (Figure 32.11) [127]. Figure 32.8 Neo‐ascending aorta (Ao) and arch reconstruction. (A) The open distal arch is interdigitated into the cutback in the proximal descending aorta and the posterior walls sutured together. A small cutback is made in the anterior aspect of the descending aorta. The connection between the cutback in the pulmonary root and the adjacent points of the proximal ascending aorta is accomplished. (B) A patch, typically a pulmonary homograft, is used to complete the arch and neo‐ascending aorta reconstruction. The patch is in the shape of a quarter circle with a radius of 3 cm. The straight edge of the patch is sutured to the inner curvature, and the curved edge is sutured to the outer curvature; the arrows indicate the alignment. (C) When the junction of the ascending aorta and pulmonary root is reached, a redundant portion of the patch is trimmed away and the proximal connection between the patch and the pulmonary root is completed. Source: [101], Tweddell JS, et.al., (2012). Reproduced with permission from Elsevier. Figure 32.9 Various methods for establishing a source of pulmonary blood flow. (A) Right ventricle (RV) to pulmonary artery (PA) shunt using Gore‐Tex tube graft. Conduit is placed on the left side of the neo‐ascending aorta. Source: [114] Sano S et al., (2012). Reproduced with permission Elsevier. (B) RV–PA shunt using Gore‐Tex tube graft with external ring: the “Dunk technique.” Conduit is placed on the right side of the neo‐ascending aorta. Source: [101], Tweddell JS, et al., (2012). Reproduced with permission from Elsevier. (C) Modified Blalock–Taussig–Thomas shunt. Conduit is connected to the center of the PA. RA, right atrium. Source: Reproduced by permission from Tweddell JS. Oper Tech Thorac Cardiovasc Surg. 2005;10:123–140. Figure 32.10 Multichannel recording of arterial saturation (SaO2), mean arterial blood pressure (MAP), and superior vena cava saturation (SvO2) during the first 4 hours after the Norwood procedure in a patient with aortic atresia. Two episodes of decreased oxygen delivery were identified with SvO2 (opposing arrows). Conventional parameters (MAP and SaO2) do not show any significant change. Conventional parameters cannot reliably provide information regarding balanced circulation. Source: [128] / with permission of Elsevier.
CHAPTER 32
Hypoplastic Left Heart Syndrome
Epidemiology
Fetal Development
Anatomy
Common Anatomic Features
Additional Anatomic Features
Clinical Features and Diagnosis
Management of the Neonate with HLHS
Initial Stabilization
Staged Reconstruction
Stage 1 Palliation: Norwood Procedure
Postoperative Management
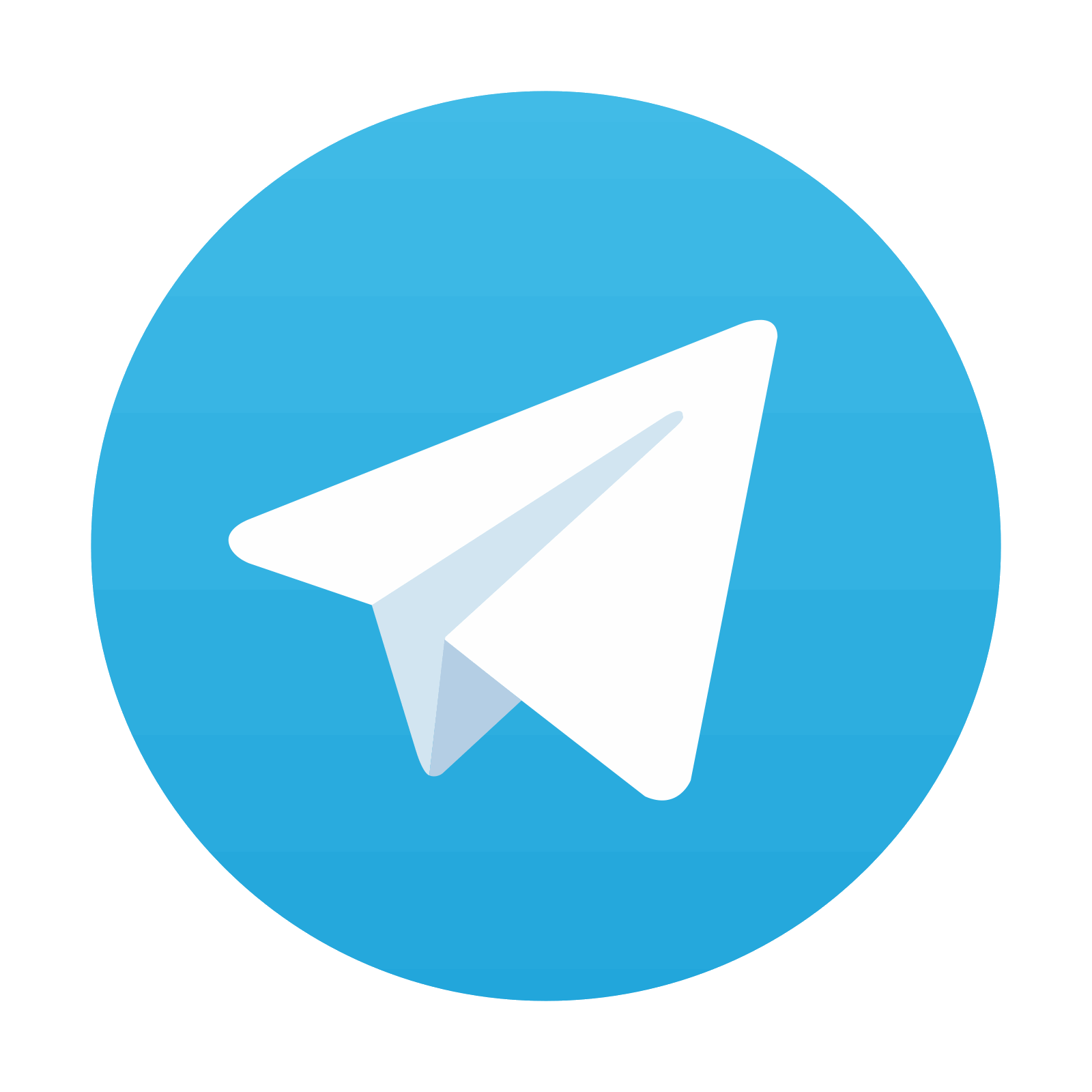
Stay updated, free articles. Join our Telegram channel
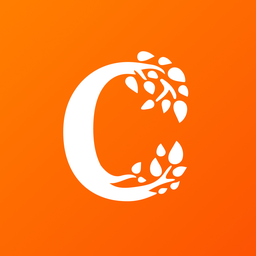
Full access? Get Clinical Tree
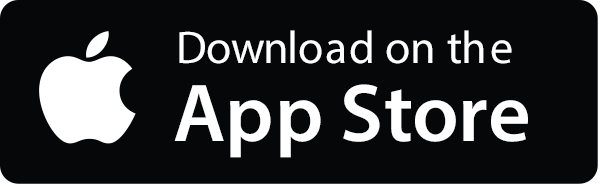
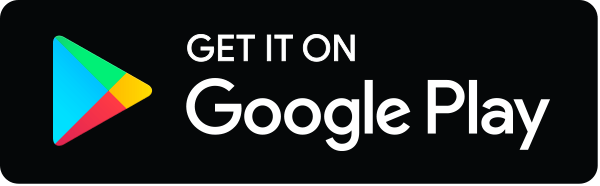