Nicholas D. Andersen, James M. Meza, and Joseph W. Turek Duke University Medical Center, Durham, NC, USA The development of cardiopulmonary bypass (CPB) and the modern era of open‐heart surgery arose from early attempts to correct intracardiac congenital heart defects in children using extracorporeal circulation. In 1952, F. John Lewis at the University of Minnesota successfully closed an atrial septal defect (ASD) without CPB using moderate hypothermia and brief periods of caval occlusion to enter the right atrium [1]. In 1953, John H. Gibbon, Jr., of the Jefferson University Medical Center, performed the first successful open‐heart surgery with the use of CPB, and closed an ASD in an 18‐year‐old female [2]. However, Gibbon was unable to replicate this success and later abandoned the technique [3]. While efforts continued to develop a safe technique for CPB, C. Walton Lillehei at the University of Minnesota described cross‐circulation from donor patients, with the initial procedure in humans performed in 1954. In Lillehei’s initial series of 45 patients, the cross‐circulation technique was again used to repair congenital heart defects such as ventricular septal defects (VSDs), atrioventricular septal defects, and tetralogy of Fallot [4]. One year later, in May 1955, John W. Kirklin and colleagues at the Mayo Clinic described a series of eight patients with congenital heart defects (five with VSDs, two with atrioventricular septal defects, and one with tetralogy of Fallot) who underwent surgery using a CPB machine similar to that previously used by Gibbon, yielding an impressive 50% survival. In the conclusion of his landmark article Kirklin declared, “Use of this system established excellent conditions for precise, unhurried intracardiac surgery” [5]. Thus, congenital heart surgeons and pediatric patients were the original pioneers of the modern age of cardiac surgery. Sequential advances in CPB technology, and careful research into the physiology and management of extracorporeal circulation over the ensuing six decades, have allowed surgeons to successfully repair complex congenital cardiac disease with low morbidity and mortality. However, astute knowledge of the principles and management of modern pediatric CPB is required to achieve optimal outcomes. The safe conduct of CPB in the neonate and infant requires a comprehensive understanding of the unique physiologic and anatomic complexities associated with extracorporeal perfusion in children with congenital heart disease (CHD). Tables 9.1 and 9.2 summarize many of the important features of pediatric CPB in relation to adult patients [6, 7]. The most important differences affecting the management and physiologic response to CPB in children include the marked hemodilution and allogenic blood product exposure associated with the pump prime, frequent use of hypothermia with low‐flow bypass or circulatory arrest, and immature organ systems. Together, these factors are believed to directly exacerbate the neuroendocrine and systemic inflammatory response and contribute to the morbidity of CPB in children. Additional anatomic challenges include placement of potentially obstructing cannulas in small vascular structures, steal from macro‐ or microcollateral vessels leading to increased left atrial blood return and/or impaired systemic perfusion [8], management of other complex intra‐ and extracardiac shunts and coronary anomalies, and extensive suture lines in patients with multifactorial coagulopathy. Table 9.1 Differences between adult and pediatric cardiopulmonary bypass. Source: Reproduced by permission from DiNardo JA, in Pediatric Cardiac Anesthesia. Philadelphia, PA: Lippincott Williams & Wilkins; 2005, p. 229. Table 9.2 Unique pediatric characteristics. Source: Reproduced by permission from Berkowitz DH, Gaynor JW, in Pediatric Cardiac Surgery, 4th ed. Chichester: Wiley‐Blackwell; 2013, pp. 169–213. CPB leads to a systemic inflammatory response that is more pronounced in children than in adults [9]. Triggers of systemic inflammation with CPB include (i) contact of blood with the foreign surface of the bypass circuit; (ii) contact of blood with air; (iii) mechanical shear stress and cellular destruction as blood passes through suction systems and filters; (iv) surgical trauma; (v) tissue ischemia and reperfusion; (vi) hemodilution and prime constituents; (vii) immune response to allogenic blood products; (viii) hypothermia; (ix) hypotension, low perfusion pressure, and nonpulsatile perfusion; and (x) protamine administration [10]. Of note is that many of these exposures are more pronounced in the pediatric population due to the increased size of the circuit relative to body surface area in children, and the increased complexities of the operation and perfusion strategy. These factors result in the activation of both humoral and cellular immune responses, which in turn activate the complement, coagulation, and fibrinolytic pathways. Collectively these pathways result in endothelial damage, capillary leak, fluid retention, and whole‐body edema [11]. Other deleterious effects include vasoconstriction, reduced organ perfusion, direct tissue injury, pulmonary hypertension, and increased pulmonary vasoreactivity [12], and up to 50% of children have pulmonary and cardiovascular dysfunction attributable to this proinflammatory response in the post‐CPB period [13]. Figure 9.1 demonstrates some of the known mediators of inflammation in response to surgical trauma and CPB. Key mechanisms include endothelial injury, complement activation, neutrophil activation and degranulation, platelet activation and aggregation, histamine release, clotting factor and fibrinolytic pathway activation, kallikrein and bradykinin activation, B1 and B2 receptor simulation, and release of nitric oxide, free radicals, eicosanoids, and a milieu of proinflammatory and anti‐inflammatory cytokines [10, 13–17]. Other mechanisms of injury and inflammation following CPB identified more recently include release of circulating micro‐RNAs and mitochondrial DNA [18–20]. Several therapeutic maneuvers have been proposed to limit inflammation from CPB, including the addition of steroids into the pump prime, heparin‐coated CPB circuitry, and ultrafiltration. These strategies have had mixed results and will be discussed in later sections. However, it is widely understood that the bypass circuit itself is probably the main culprit in inciting the inflammatory cascade. In pediatric cardiac surgery, circuit and hardware design therefore require significantly greater scrutiny than in adult perfusion systems. Other aspects of the perfusion plan that can impact the inflammatory response and require close attention include use of cardiotomy suction, temperature management, flow rates, perfusion pressures, regional perfusion strategies, hemodilution, hematocrit levels, transfusion strategy, and blood gas strategy. Thus, nearly all aspects of the perfusion plan must be carefully analyzed and coordinated in order to provide the safest interaction between the patient and the extracorporeal circulation system. Finally, despite the increased safety of CPB in the modern era, it should be recognized that exposure of children to CPB remains a major source of morbidity following heart surgery, and the surgeon should aim to perform an efficient operation with judicious use of CPB when possible. Figure 9.1 Illustration of effects of surgical trauma and cardiopulmonary bypass (CPB). Schematic representation of important mediators of the inflammatory response to surgical trauma and CPB. The different processes have a synergistic effect and may lead to organ dysfunction. IL, interleukin; MPO, myeloperoxidase; NO, nitric oxide; PAF, platelet activating factor; PMN, polymorphonuclear neutrophils; TNF, tumor necrosis factor. Source: Reproduced with permission from Brix‐Christensen V. Acta Anaesthesiol Scand. 2001;45:671–679. The goal of CPB is to provide adequate oxygen delivery to the tissues while the heart is unable to support the circulation. Monitoring the adequacy of perfusion and oxygen delivery can be achieved using several direct and indirect measurements, summarized in Table 9.3. Perfusion pressure is generally maintained at a lower state in children due to the lower baseline blood pressure, lack of atheromatous disease and acquired cardiovascular stenoses, and the use of vasodilation to achieve uniform cooling and rewarming [21]. The mean arterial pressure (MAP) is also maintained within the autoregulatory range for cerebral blood flow, generally 30–45 mmHg for neonates and 40–60 mmHg in infants [22]. Cerebral perfusion pressure (CPP) is the difference between MAP and central venous pressure (CVP), and measurement of CVP from an upper‐body central venous catheter helps to ensure the adequacy of CPP and cerebral venous drainage. A normal cardiac index typically ranges from 2.5 to 4 L/min/m2, and by convention “full‐flow” CPB is generally around 3 L/min/m2. However, safe flow rates for adequate oxygen delivery are affected by hematocrit, temperature, and pH management strategy [23]. It should be noted that in the setting of steal from aortopulmonary collaterals, perfusion pressure and the effective cardiac output may be significantly reduced [24]. In instances where shunts or collateral vessels cannot be controlled, increased flow rates may be required. Alternatively, hypothermia is commonly employed to reduce metabolic oxygen requirements and allows for lower flow rates (Table 9.4). Table 9.3 Measures of adequate perfusion on cardiopulmonary bypass. Table 9.4 Common index flows during cardiopulmonary bypass. Source: Reproduced by permission from Matte GS, in Perfusion for Congenital Heart Surgery. Hoboken, NJ: Wiley‐Blackwell; 2015, pp. 33–71. Monitoring the oxygen saturation of venous blood (SvO2) can provide some reassurance of the adequacy of oxygen delivery, and is generally maintained at 70% or greater at normothermia. However, shunting may artificially elevate the SvO2 as oxygenated blood recirculates through the lungs, to the left atrium, and back to the venous reservoir via intracardiac shunts or vents. At low temperatures the SvO2 also becomes less reliable, as hypothermia causes oxygen to become more tightly bound to hemoglobin. SvO2 values of 90% or greater may be observed during deep hypothermia. Other important measures of the adequacy of oxygen delivery include arterial blood gas values and lactate levels on CPB [25]. Near‐infrared spectroscopy (NIRS) is also commonly used to assess cerebral perfusion and oxygen delivery, and provides an assessment of venous oxygen saturation levels in the brain at a depth of 1–2 cm. NIRS values have been shown to correlate with superior caval vein (SVC) oxygen saturations and neurodevelopmental outcomes [26, 27], and have been used to predict the safe duration of circulatory arrest [28]. Blood contact with air or the nonendothelial surface of the bypass circuit will result in activation of the clotting cascade and thrombus formation within the patient and circuit, potentially causing circuit failure, embolism, stroke, consumptive coagulopathy, or death. Thus, therapeutic anticoagulation is vital to prevent the formation of clot while on CPB. The anticoagulant universally used in both adult and pediatric CPB is heparin. Heparin dosing is typically between 300 and 400 IU/kg. The goal activated clotting time (ACT) is ≥480 seconds. This is based on early work by Bull and colleagues, who formulated a heparin–protamine dose–response curve in the attempt to avert the use of either excess heparin or protamine [29]. Heparin works by binding to antithrombin III (ATIII), resulting in a conformational change in the enzyme and enhancing its activity by a factor of a thousand. ATIII functions to inactivate thrombin and other proteases, most notably Factor Xa, thus preventing ongoing propagation of the clotting cascade. The level of ATIII is decreased in neonates and will only reach adult levels by 3–6 months of age. Patients in this age group may be at risk for inadequate anticoagulation, and fresh plasma or ATIII concentrate may be required to achieve an adequate ACT in patients with ATIII deficiency. Studies have shown that despite an adequate ACT and lack of visible clotting, there may be thrombin generation at a cellular level, which may lead to increased platelet binding, decreased levels of factor VIII, and consumptive coagulopathy. Based on these factors, investigators have suggested that a protocol based on heparin concentration may be more effective [30–32]. A study by Guzzetta and colleagues found that use of the heparin concentration technique led to decreased thrombin generation as well as preserved factor VIII levels, suggesting improved anticoagulation. A randomized controlled trial from Toronto also reported a reduced need for transfusions and improved postoperative outcomes in children under 1 year of age when using heparin concentration measurements for anticoagulation management with CPB [30]. A concern in patients presenting for repeat cardiac surgery is the incidence of heparin‐induced thrombocytopenia (HIT). HIT is an immunologic response to heparin therapy with platelet‐activating, anti‐platelet factor 4 (PF4)/heparin antibodies leading to thrombocytopenia associated with thromboembolism [33]. In the adult cardiac experience, the quoted incidence of HIT post CPB is 1–3%, with a thrombotic morbidity of between 38% and 81% and a mortality of 28% [34]. The presence of anti‐PF4/heparin antibodies occurs in up to 60% of adult cardiac surgery patients, but only the quoted 1–3% will develop clinical HIT with thrombocytopenia and thrombosis [33]. In a series of reoperative pediatric heart surgery patients, a similar incidence of a 50% positive antibody screen was detected 10 days postoperatively [35]. Further, in a case series and literature review of neonates, infants, and young children, the authors concluded that the incidence of HIT with associated thrombotic complications (∼1.3%) was underappreciated and likely equal to that in the adult population [36]. What are the alternatives to the use of heparin in a pediatric patient with a history of HIT? If anti‐PF4/heparin antibodies are undetectable and platelet counts have returned to normal, then heparin re‐exposure is permissible [37]. For patients with ongoing seropositivity or acute clinical HIT, options include (i) the use of an alternative anticoagulant with the complete avoidance of all heparin both intra‐ and postoperatively; (ii) standard heparin anticoagulation with a platelet antagonist; and (iii) plasmapheresis followed by heparin exposure [37]. Although all strategies have been used successfully, the current American College of Chest Physicians recommendation is to use the direct thrombin inhibitor (DTI) bivalirudin for the procedure and postoperative period, because of the evidence demonstrating its usefulness and safety compared with other DTIs [37–39]. At the termination of CPB, protamine will be administered to reverse the anticoagulation effect of heparin. Protamine dosing can be empiric, usually in a 1 : 1 ratio with heparin (or 3–4 mg protamine/kg), or can be calculated using a heparin dose–response curve [22]. In adult cardiac surgery severe adverse reactions to the administration of protamine occur in up to 2.6% of patients and include anaphylactic and anaphylactoid reactions as well as pulmonary hypertensive events [40]. Pediatric patients, however, have different risks as they have less frequent or shorter exposures to cross‐reacting antigens and lower sensitivity of pulmonary intravascular macrophages to heparin–protamine complexes [41]. A study of the incidence of protamine reaction in 1249 pediatric patients ≤16 years of age found that the incidence of hypotension that persisted for >5 minutes was 1.76%. Risk factors for hypotension included female sex, large dose of protamine, and small heparin dose [41]. Thus, patients should be carefully monitored for adverse events after protamine exposure and administration over 5–10 minutes may help reduce the incidence of hypotension [22]. Allogenic blood product transfusion leads to inflammation [42], allosensitization [43], and a small risk of blood‐borne infections and anaphylactoid reactions. However, the risk of blood product transfusion must be weighed against the risk of inadequate oxygen delivery as a result of hemodilution from the pump prime. The hematocrit value during bypass is directly proportional to the preoperative hematocrit and inversely proportional to the prime volume (Figure 9.2) [44]. These considerations become more important in infants and neonates, where the volume of the pump prime may exceed the circulating blood volume. Traditionally, hematocrit levels below 20% were considered safe [45, 46] and perhaps even advantageous, as they are associated with fewer transfusions and may lead to improved microvascular oxygen delivery during hypothermia as a result of lower blood viscosity [47, 48]. However, the Boston hematocrit trials presented randomized data on infants undergoing low‐flow CPB with hypothermia. The first study randomized patients to a target hematocrit level of 20% versus 30% and found that patients randomized to the lower hematocrit group had worse outcomes, including lower nadir cardiac index, higher serum lactate levels, and a greater increase in total body water. At 1 year of age this same group had lower Psychomotor Development Index scores [49]. In a second study, similar patients were randomized to hematocrit levels of 25% versus 35% on CPB, and results showed no significant differences in perioperative or neurodevelopmental outcomes at 1 year between groups [50]. In a third study, data from the two trials were combined and hematocrit level on CPB was analyzed as a continuous variable. In this analysis, a hematocrit level of approximately 24% or higher was associated with higher Psychomotor Development Index scores and reduced lactate levels [51]. As a result, in current practice most centers aim to maintain a hematocrit level between 25% and 30% while on CPB through a combination of banked blood transfusion and ultrafiltration. Transfusion‐free cardiac surgery in neonates and infants has been achieved with the use of microcircuits and reduced prime volumes, but typically at the expense of lower hematocrit targets [44, 52–60]. Figure 9.2 Hemodilution with asanguinous prime for 3 kg neonate. BV, biventricular; Hct, hematocrit; pat, paroxysmal atrial tachycardia. Source: Reproduced with permission from Golab HD et al. Perfusion. 2010;25:237–243. pH and PCO2 levels vary with temperature and interact with the cerebral vasculature. Management priorities for pH and PCO2 with hypothermic CPB differ between children and adults, primarily due to differences in the presumed mechanisms of neurologic injury. In adults, embolic phenomena are believed to be the primary source of neurologic injury, suggesting that limiting cerebral blood flow and the delivery of emboli may be preferable. In children, brain injury is believed to be due to inadequate cerebral blood flow and oxygen delivery under conditions of low‐flow bypass or circulatory arrest. Thus, strategies that lead to increased cerebral blood flow, homogenous brain cooling, and reduced cerebral metabolic rate of oxygen (CMRO2) may be preferable. Hypothermia leads to an alkaline (increased) pH and decreased PCO2 as a result of decreased dissociation of H+ ions and increased solubility of carbon dioxide in liquids (Figure 9.3) [61]. The “alpha‐stat” blood gas management approach, used more commonly in adults, aims to maintain the PCO2 at 40 mmHg and the pH at 7.40 when measured at 37 °C, regardless of body temperature [22]. In essence, blood pH levels in the hypothermic patient are allowed to rise naturally, and PCO2 levels are allowed to fall naturally, as long as pH and PCO2 levels are in the normal range when analyzed at normothermia. This strategy is intended to maintain intracellular electrochemical neutrality with maintenance of normal enzymatic function by maintaining a constant hydrogen ion concentration gradient between the intracellular and extracellular environments at both normothermia and hypothermia. The strategy is termed alpha‐stat, as the ratio of dissociated to nondissociated imidazole (alpha) groups on histidine, the dominant intracellular protein buffer, remains constant [62]. Maintenance of normal intracellular enzymatic activity is intended to maintain the function of autoregulatory systems in the cerebral vasculature, where blood flow is matched to metabolic need. Simply put, this strategy intends to achieve just the right amount of cerebral blood flow to meet metabolic needs but not excessive cerebral blood flow, which could theoretically increase embolic events. However, it should be noted that at deep hypothermic temperatures of 22 °C or less, cerebral autoregulation is lost [63, 64]. Figure 9.3 The effect of temperature on blood plasma pCO2 (A) and pH (B) comparing the alpha‐stat and pH‐stat protocols of acid–base management. Source: Reproduced with permission from Tallman RD Jr. J Cardiothorac Vasc Anesth. 1997;11:282–288. In children, strategies that lead to increased cerebral blood flow may be preferable given the reduced risk of neurologic injury from atheroemboli and increased risk of injury from inadequate oxygen delivery. The pH‐stat strategy aims to achieve these goals. With pH‐stat, blood pH is maintained at 7.40 at all temperatures (Figure 9.3), requiring carbon dioxide to be added to the system in order to combat the natural increase in pH and decrease in PCO2 that occur with hypothermia. The purported benefits of this system are that the added carbon dioxide shifts the oxyhemoglobin dissociation curve to the right, which counteracts the left shift caused by hypothermia, and leads to increased release of oxygen to the tissues. Carbon dioxide is also a powerful cerebral vasodilator and pulmonary vasoconstrictor. This may lead to improved cerebral blood flow with improved cooling of the brain [65], as well as decreased runoff to the pulmonary circulation in the setting of shunts or aortopulmonary collateral blood flow. The lower blood pH at hypothermia may also suppress the CMRO2, leading to decreased oxygen consumption [66]. In pediatric patients, reports of choreoathetosis with alpha‐stat management [67, 68], as well as data demonstrating improved perioperative outcomes and developmental scores with pH‐stat management [69–71], have led most centers to favor pH‐stat for pediatric CPB. pH‐stat is especially preferred when low‐flow bypass or hypothermia is to be employed, or in patients with cyanotic heart disease and presumed aortopulmonary collateral burden, where increased pulmonary vascular resistance may reduce runoff to collaterals [72]. Other centers employ a cross‐over strategy where pH‐stat is used during cooling and alpha‐stat is used during rewarming [73]. Hyperoxygenation with use of 100% FiO2 to achieve PaO2 levels between 300 and 700 mmHg is currently employed by most centers for oxygen management during pediatric CPB [22]. Hyperoxygenation may lead to reduced gaseous microemboli by removing the nitrogen from the sweep gas [74], and improve oxygen delivery by increasing the quantity of dissolved oxygen, which is preferentially used by the brain during hypothermia [75]. Studies have supported the notion that hyperoxygenation leads to reduced hypoxic brain injury with circulatory arrest [76] and better attenuation of anaerobic metabolism with less acidosis [77]. However, hyperoxia also leads to increased oxygen free radical production, which may exacerbate ischemia‐reperfusion injury of the myocardium [78, 79]. The benefits of hyperoxia for neuroprotection may supersede these concerns [22]. Hypothermia is commonly used during pediatric CPB to decrease metabolism and oxygen consumption, allowing for improved end‐organ protection from ischemia during the period of extracorporeal perfusion. As temperature is lowered, cellular metabolism is reduced, and the rate of adenosine triphosphate consumption is decreased. Whole‐body oxygen consumption decreases directly with body temperature (Figure 9.4) [7, 80–82] and the CMRO2 falls to below 80% of baseline at 18 °C [64, 83]. The Q10 is defined as the ratio of metabolic rates at two temperatures separated by 10 °C. The Q10 in the pediatric population (3.65) has been found to exceed that of adults (2.4–2.8), suggesting that there is a greater decrease in metabolic rate with hypothermia in neonates, infants, and children compared to adults [64]. Although deep hypothermia (18–22 °C) was traditionally believed to provide the greatest degree of neurologic protection from ischemia, several alterations to cerebral physiology occur at these temperatures that warrant consideration. At deep hypothermia, cerebral blood flow (CBF) autoregulation is lost and CBF becomes proportional to MAP (Figure 9.5) [7, 64]. However, in some patients CBF may be lost altogether during low‐flow bypass at deep hypothermia, due to disrupted autoregulation, the development of critical closing and opening pressures, and a no‐reflow phenomenon [84–86]. Alternatively, cellular metabolism and oxygen requirements are sufficiently reduced during deep hypothermia to allow extended periods of neurologic and end‐organ protection in the complete absence of pump flow (Figure 9.6) [7, 87]. However, deep hypothermic circulatory arrest (DHCA) may lead to persistent derangements in CBF autoregulatory mechanisms, leading to reduced CBF for up to 40 hours after rewarming [63, 84, 88]. Another variable related to temperature management with DHCA is the rate of cooling and rewarming. Rapid cooling may lead to heterogenous cooling of brain regions, and worse neurologic and developmental outcomes have been observed with cooling times less than 20 minutes [67, 89]. Rapid rewarming and overwarming can also predispose to neurologic injury [90–92]. Figure 9.4 Whole‐body oxygen consumption (VO2) as a function of body temperature. Figure 9.5 Data obtained from a human infant demonstrate that cerebral autoregulation is lost at deep hypothermic temperatures (<22 °C), with cerebral blood flow (CBF) related in a linear manner to mean arterial pressure (MAP). Source: Reproduced with permission from Greeley WJ et al. J Thorac Cardiovasc Surg. 1991;101:783–794. Figure 9.6 The probability of safe circulatory arrest is related to the duration of arrest and the temperature at which it occurs. Source: Reproduced with permission from Kirklin JW, Barratt‐Boyes BG, Cardiac Surgery, 2nd ed. New York: Churchill Livingstone; 1993. From the standpoint of the surgeon, hypothermia allows for a safe reduction in pump flow rates (Table 9.3) and improved visibility due to decreased blood return to the surgical field. During DHCA, cannulas can be removed altogether and surgery can be performed in a completely uncluttered and bloodless field. Hypothermia is further used to provide a critical safety net against intraoperative misadventures where pump flow may be temporarily lost, such as airlock, massive air embolus, circuit failure or thrombosis, or cannula dislodgment [93]. Systemic hypothermia also improves myocardial protection by preventing radiant rewarming of the heart from surrounding tissues, or from warm blood return to the heart from collaterals or partial bypass [94]. Reduction in flow rates at hypothermic temperatures has also been suggested to result in decreased activation of the inflammatory cascade and gaseous microemboli due to decreased cardiotomy sucker and vent return, with decreased damage to formed cellular elements, decreased blood exposure to air, and reduced exposure of blood to the nonendothelial surfaces of the circuit [95]. However, the benefits of routine hypothermia have recently been questioned, and several centers now favor use of normothermia or mild hypothermia with congenital heart surgery when possible [96–98]. Potential disadvantages of hypothermia include increased time spent cooling and rewarming, platelet dysfunction and coagulopathy with increased transfusion requirements [99, 100], loss of cerebral autoregulation [64], and cold‐induced damage to cellular membranes and enzymatic processes, resulting in direct end‐organ injury and increased length‐ of stay [98, 101]. Several studies have failed to show a reduction in inflammatory markers with use of hypothermia [102–105], challenging previously held notions that hypothermia reduced inflammation on CPB. In addition, small randomized studies have shown reductions in inotrope requirements, transfusions, mechanical ventilation time, and length of stay with the use of mild hypothermia or normothermia [106, 107]. The superiority of normothermia has yet to be shown in any large studies or meta‐analyses [108–110], although the trend toward use of more “physiologic” bypass conditions appears to be accelerating [111]. DHCA was historically used by congenital heart surgeons to allow for complex intracardiac repairs in an uncluttered, bloodless field. The safe period of DHCA is dependent upon many factors, but multiple studies have suggested worse neurodevelopmental outcomes as DHCA time increases above 35–40 minutes (Figure 9.6) [87, 112–114]. In an attempt to reduce the period of circulatory arrest to the brain and other important vascular beds, regional perfusion strategies have been employed to provide continuous perfusion of the cerebral, splanchnic, and even coronary circulations throughout the operation. Although no concrete evidence clearly proves the superiority of these techniques relative to DHCA [115, 116], they are gaining in popularity due to the theoretical benefits of decreased ischemic time and end‐organ injury, less time spent on bypass cooling and rewarming, and avoidance of coagulopathy and other negative effects associated with deep hypothermia [98, 117]. Common regional perfusion strategies used during arch reconstruction include antegrade cerebral perfusion (or regional cerebral perfusion [RCP]), performed by direct cannulation of the innominate artery or by cannulation of an innominate artery side graft, followed by control of the brachiocephalic vessels [118]. Regional cerebral‐coronary perfusion is similar, but instead the transverse aortic arch is clamped after the innominate artery takeoff, allowing for continuous flow to the heart and brain during distal arch reconstruction. Visceral perfusion during arch reconstruction can be combined with these techniques with the use of a descending aorta arterial cannula (Figure 9.7) [119–121]. The most aggressive regional perfusion strategy during arch surgery involves three‐region perfusion of the heart, brain, and viscera, by use of a visceral perfusion cannula, as well as continuous coronary perfusion with an ascending aorta cardioplegia needle or olive‐tipped cardioplegia catheter (Figure 9.8). Our group has recently transitioned to a three‐region beating‐heart perfusion technique with continuous coronary perfusion performed under mild hypothermia, for arch surgery and the Norwood reconstruction, with favorable results [122–124]. Studies have shown that these techniques may lead to reduced end‐organ injury, a larger “safety net” for attention to technical detail, reduced bypass times, and operation at warmer temperatures [119, 121–126]. Figure 9.7 Schematic depiction of three‐region perfusion strategy during neoaortic arch reconstruction. Shaded bars depict the timeline of direct perfusion (shaded) or ischemia (white) to the cerebral (brain), splanchnic (kidney), and coronary (heart) circulations. Upper panel: three‐region perfusion strategy described in this chapter, with drawings (A), (B), and (C) representing the corresponding stages of surgery. (A) The cerebral and coronary circulations are perfused throughout the distal arch reconstruction. (B) Distal arch complete, direct perfusion of the splanchnic circulation is resumed. (C) Cardioplegia is administered and the coronary circulation is interrupted during only the proximal neoaortic arch completion. The direct perfusion of the cerebral circulation is uninterrupted throughout. Lower panel: (D) perfusion and ischemia timeline of the standard approach, with continuous cerebral perfusion throughout, but cardioplegia and interruption of the coronary and splanchnic circulations during the entire neoaortic arch reconstruction. Typically, this results with corresponding cooling, rewarming, and overall cardiopulmonary bypass time longer than that of the three‐region strategy. Source: Reproduced by permission of Elsevier from Karavas AN et al. Ann Thorac Surg. 2011;92:1138–1140. Figure 9.8 Illustrations of cerebral and coronary perfusion strategies used during beating‐heart Norwood neoaortic reconstruction. (A) Continuous cerebral and coronary perfusion is provided by a 3.5 or 4 mm graft sewn onto the innominate artery. Selective clamping of the descending aorta, left subclavian artery, left common carotid artery, and proximal aortic arch enables aortic arch augmentation. For the ascending aortic reconstruction, only antegrade cerebral perfusion continues to be supplied through the innominate artery graft because a clamp is placed on the proximal vessel. Concurrently, continuous coronary perfusion is maintained through an accessory cannula placed in the ascending aorta proximal to the augmentation. (B) A cardioplegia spike can be used for larger‐diameter aortas with a clamp placed just distally to the spike. (C) For smaller ascending aortic diameters, an olive‐tip cannula is placed into the lumen of the opened aorta and directed toward the root. A vessel loop tourniquet can be used to secure the cannula. Source: Reproduced by permission of Elsevier from Turek JW et al. Ann Thorac Surg. 2013;96:219–224. Ultrafiltration involves passage of blood through the semipermeable membrane of a hemoconcentrator to remove water, electrolytes, and other small molecules less than the pore size of the filter. The goals of ultrafiltration during CPB include removal of excess crystalloid volume, hemoconcentration to increase the hematocrit and reduce the need for blood transfusions, and removal of electrolytes and inflammatory mediators. These maneuvers are of greater priority in neonates and infants due to their increased susceptibility to hemodilution, transfusions, and inflammation associated with CPB. Ultrafiltration can be performed before, during, and/or after bypass. Pre‐bypass ultrafiltration (PBUF), also called prime ultrafiltration (PUF), is used to adjust the electrolyte levels of blood‐primed circuits prior to the initiation of bypass. The objective of PBUF is to remove potassium, lactate, glucose, and other harmful preservatives and byproducts associated with banked blood. The discarded ultrafiltrate is replaced with a balanced electrolyte solution in order to achieve a physiologic prime solution. Decreased inflammatory mediators and improved clinical outcomes have been shown with the use of PBUF techniques in small studies [127]. Ultrafiltration during bypass, termed conventional ultrafiltration (CUF), aims to remove excess fluid that accumulates during CPB from multiple sources (pre‐bypass fluid administered to the patient, cardioplegia, valve testing solution, crystalloid added to the venous reservoir during periods of decreased venous return, etc.) [22]. In zero‐balance ultrafiltration (ZBUF), or dilutional ultrafiltration (DUF), ultrafiltrate is continually removed and replaced with an equivalent volume of crystalloid, to achieve a zero net fluid balance. Here, the goal is to continuously remove electrolytes (potassium, lactate, etc.) and inflammatory mediators to produce a circulating volume with a constituency similar to that of the crystalloid replacement solution. Studies of ZBUF techniques have shown decreased levels of inflammatory mediators and modest improvements in postoperative pulmonary function in pediatric patients [128–130]. Ultrafiltration performed after bypass, termed modified ultrafiltration (MUF), was first described in 1991 by Naik and colleagues and was intended to remove excess body water, salvage residual bypass circuit blood, increase the hematocrit, and decrease transfusions [131]. Additional purported benefits of MUF later reported by these and other authors include increased blood pressure, decreased inotropic requirements, reduced levels of inflammatory mediators, decreased myocardial edema with improved left and right heart function, improved coagulation parameters, improved pulmonary function, decreased postoperative effusions, and decreased length of intensive care unit (ICU) stay [132–141]. However, the ancillary benefits of MUF above and beyond hemoconcentration, blood salvage, and increased blood pressure (in part due to increased blood viscosity) have not been universally established [142–144]. The standard technique for MUF is shown in Figure 9.9. A clamp is applied that disconnects the venous line from the venous reservoir. A line is then brought up to connect the ultrafilter directly onto the venous line. The direction of flow to the ultrafilter is from the venous reservoir and from the arterial line. A roller pump is interposed between the blood source and the filter to ensure that blood moves toward the patient at a rate of approximately 200 mL/min. Suction is also applied to the filter at about –125 mmHg to achieve a filter rate of approximately 100–150 mL/min [134]. Alternative configurations for MUF exist, and primarily differ between whether the arterial line or the venous line is used to supply blood from the patient to the MUF circuit [145]. The phases of MUF include normovolemic, hypovolemic, and transfusion. During the normovolemic phase ultrafiltrate is replaced with blood from the venous reservoir. Once the venous reservoir blood has been exhausted, the hypovolemic phase entails hemoconcentration of the patient without replacement of the ultrafiltered volume. Filling pressures fall until a “safe” but lower filling and blood pressure are achieved. The transfusion phase then entails simply flushing the MUF circuit volume forward in order to transfuse the patient back to the desired filling pressure [22]. Figure 9.9 Set‐up for modified ultrafiltration. Source: Reproduced by permission from Davies MJ et al. J Thorac Cardiovasc Surg. 1998;115:361–370. Disadvantages of MUF include increased time spent heparinized at the conclusion of the procedure with a potential for worsening coagulopathy, increased procedure time, inadvertent cooling (or excessive rewarming) depending on how the patient’s temperature is managed, hypotension or coronary insufficiency if excessive volume is removed from the arterial line, air entrainment, or circuit complications related to the technical complexity of the procedure. Further, the reduced prime volumes of modern circuits and routine use of conventional ultrafiltration to achieve higher hematocrit targets on bypass have led some centers to abandon the technique, without a detriment to patient outcomes [146]. In summary, ultrafiltration techniques are important adjuncts to the pediatric CPB plan to achieve hemoconcentration and reduce the need for transfusions, but the specific protocols and techniques used vary widely between programs and are often dictated by institutional expertise. Bleeding after separation from CPB represents a ubiquitous and vexing problem in congenital cardiac surgery, which can significantly impact patient outcomes and the postoperative trajectory. Prolonged, uncontrolled bleeding leads to hemodynamic instability and increased exposure to allogenic blood products. This in turn can exacerbate the systemic inflammatory response; promote end‐organ injury, fluid retention, and pulmonary dysfunction; and prolong the postoperative recovery. Ongoing bleeding in the ICU can further lead to hemodynamic instability, low cardiac output syndrome, increased inotrope requirements, end‐organ injury, tamponade, cardiac arrest, extracorporeal membrane oxygenation (ECMO), or even death. Thus, development of a reliable protocol for correction of coagulopathy and disciplined control of hemostasis after CPB is mandatory for optimal outcomes. The origins of post‐bypass coagulopathy in pediatric patients are multifactorial and include preoperative and intraoperative factors. At birth, concentrations of the vitamin K‐dependent clotting factors (II, VII, IX, X), contact factors (XI and XII), and anticoagulant and inhibitory factors (antithrombin III, protein C, protein S, CI esterase inhibitor, and plasminogen) are all reduced, making the coagulation system more susceptible to hemodilution [147, 148]. Cyanotic patients are further known to have impaired coagulation due to polycythemia, abnormal platelet count and function, decreased factor concentration (V, VII, and VIII), and increased fibrinolysis [148, 149]. Additional preoperative factors associated with coagulopathy in neonates and infants are shown in Table 9.5, and include platelet dysfunction from prostaglandin administration, hepatic dysfunction from lesions with high systemic venous pressures, and von Willebrand factor degradation from lesions resulting in high shear forces [12]. Intraoperative factors contributing to coagulopathy are primarily related to the conduct of CPB, and include hemodilution, hypothermia, platelet activation, clotting factor consumption, and destruction of formed elements from cardiotomy suction, line filters, and exposure to tubing and air. As a result, judicious use of CPB and operation at warmer temperatures are viewed by many as the simplest ways to limit the deleterious effects of CPB on coagulopathy. Technical factors such as suturing technique and patch material are also heavily scrutinized by surgeons and are often blamed for admirable or poor hemostasis. Table 9.5 Factors related to coagulopathic complications in neonates and infants. Source: Reproduced by permission from Jaggers J, Lawson JH. Ann Thorac Surg. 2006;81:S2360–S2366. Restoration of coagulation and control of bleeding after CPB can be achieved using a myriad of approaches, and most centers have adopted standardized protocols for measuring coagulation parameters and directing transfusion efforts. First and foremost, surgical hemostasis should be achieved by careful inspection of cannulation sites and suture lines to identify and correct areas of active bleeding above and beyond needle holes. After protamine administration, packing with sponges and/or topical hemostatic agents such as Gelfoam soaked in thrombin or fibrin sealants is then commonly performed. Further assessment of coagulation system function can be made by measuring the ACT, heparin level, platelet count, fibrinogen level, prothrombin time, partial thromboplastin time, and/or thromboelastography [150, 151]. Post‐bypass transfusions are normally first centered on repletion of platelets, given the significant reduction in platelet quantity and function after bypass, as well as cryoprecipitate to normalize fibrinogen and clotting factor levels [152]. These transfusions can be administered empirically or as part of a goal‐directed protocol based on the results of laboratory tests. Other commonly employed adjuncts include antifibrinolytics [147, 153, 154], activated recombinant factor VII [155], fibrinogen concentrate [156], and prothrombin complex concentrates [157, 158]. However, restraint should be exercised with use of the more powerful adjuncts, such as factor VII [159], as thrombotic complications from intrinsic hypercoagulability or overcorrection of the coagulation system generally predominate over the remainder of the postoperative course [160–162]. The precise methodology by which various laboratory assays, transfusions, adjuncts, and hemostatic products are selected and integrated into a comprehensive hemostasis plan varies from center to center, and is further dependent on the specific surgeon and perfusion factors that may predispose to more or less bleeding. One final topic of debate is whether priming the pump with fresh (<48 hours old) whole blood, or reconstituted fresh whole blood from a single donor, leads to improved hemostasis after CPB. Although results are conflicting, some studies have shown that hemostasis and postoperative outcomes may be improved in neonates with the use of fresh whole blood preparations, due to improved storage conditions, younger age of cellular components, improved platelet and coagulation factor function, and/or reduced donor exposures [163–166]. At the initiation of CPB, arterial line pressures, venous drainage, and flow rates are assessed to ensure proper cannula position and function. When full‐flow bypass is achieved, ventilation will cease and the adequacy of perfusion will be monitored as described above. Large collateral vessels (such as the arterial duct or pre‐existing surgical shunts) should be quickly controlled or occluded to prevent runoff to the pulmonary circulation, low perfusion pressures, and inadequate systemic perfusion. Vents are then placed, and the patient is cooled to the desired temperature coincident with the surgical plan. After completion of the repair, the patient is rewarmed to normothermia or mild hyperthermia [92], taking care to avoid injury from rapid rewarming or overwarming [90, 91, 167]. An adequate cardiac rhythm must be established and augmented by epicardial pacing if necessary. The use of inotropic support will depend on the case, the presence of pre‐existing myocardial dysfunction, and the duration of CPB. In the pediatric setting this may take the form of dopamine, epinephrine, and milrinone, but under certain circumstances vasopressin may be necessary. Concomitant with this will be the suctioning and re‐expansion of the lungs and resumption of ventilation. It is important that the lungs are fully re‐expanded as atelectasis will lead to hypoxemia and elevated pulmonary artery pressures from direct compression of the pulmonary bed. In the neonate with reactive pulmonary vasculature this may lead to a spiral of increasing hypoxia, worsening pulmonary hypertension, and right ventricular failure, which may necessitate return to bypass. The principles of preventing this phenomenon include adequate ventilation and gas exchange, avoiding acidosis, use of the phosphodiesterase inhibitor milrinone, and, occasionally, inhaled nitric oxide. Intraoperative echocardiography (transesophageal or epicardial) is vital in all patients after corrective congenital heart surgery prior to separation from bypass. Echocardiography is used to confirm the success of de‐airing maneuvers and confirm acceptable ventricular function without regional wall motion abnormalities suggestive of unrecognized coronary ischemia. Once the preliminary echocardiography exam is complete, the heart is gradually filled, and the patient is weaned from bypass. A detailed echocardiography exam is repeated to ensure an anatomically sound repair without important residual lesions that could portend failure or reintervention. MUF is performed if desired, followed by protamine administration and removal of cannulas. The use of smaller circuits and prime volume is aimed at decreasing hemodilution and decreasing the total foreign surface area with which the smaller circulating volume of the pediatric patient will make contact. In theory this will result in lower numbers of transfusions, decreased severity of the inflammatory response, and thus reduced length of stay and improved outcomes [44, 52, 54–56, 59
CHAPTER 9
Management of Pediatric Cardiopulmonary Bypass
History
Principles of Pediatric Cardiopulmonary Bypass
Special Considerations for Pediatric Patients
Parameter
Adult
Pediatrics
Hypothermic temperature
Rarely <25–32 °C
Commonly 15–20 °C
Circulatory arrest
Uncommon
Common
Pump prime
Dilutional effect on blood volume
25–33%
200–300%
Additional additives
Blood, albumin
Perfusion pressure
50–80 mmHg
20–50 mmHg
Influence of pH management
Minimal at moderate hypothermia
Marked at deep hypothermia
Measured PaCO2 differences
30–45 mmHg
20–80 mmHg
Glucose regulation
Hypoglycemia
Rare – requires liver injury
Common – ↓ glycogen stores
Hyperglycemia
Frequent – insulin bolus or infusion
Less common – rebound ↓↓ glucose
Smaller circulating blood volume
Higher oxygen consumption
Reactive pulmonary vascular bed
Intra‐ and extracardiac shunting
Altered thermoregulation
Poor tolerance for microemboli
Systemic Inflammatory Response to Cardiopulmonary Bypass
Monitoring
Direct measures
Mean arterial blood pressure generated by pump
Cardiac index generated by pump
SVO2
Cerebral oximetry
Jugular bulb saturation
Indirect measures
Acid–base status
Plasma lactate
Urine output
Patient temperature (°C)
Bypass flow rate (L/min/m2)
≥35
2.4–3.5
32
2.2
30
2.0
28
1.8
26
1.6
24
1.4
22
1.2
20
1.0
<20
0.7
Anticoagulation and Reversal
Hemodilution and Hematocrit
Blood Gas Management
Temperature
Regional Perfusion Strategies
Ultrafiltration
Management of Post‐Bypass Coagulopathy
Procedure‐independent factors
Procedure‐dependent factors
Ductal‐dependent circulation
Hemodilution
Prostaglandin administration
Hypothermia
Cyanosis
Circulatory arrest
Prematurity
Redo operation
Immature clotting systems
Prolonged cardiopulmonary bypass
Low cardiac output
Use of prosthetic material
Neurologic injury
Low cardiac output
Sepsis
Altered physiology
Malnutrition
Hepatic dysfunction
Shear forces
Initiation and Termination of Cardiopulmonary Bypass
Circuit Considerations for Pediatric Patients
Circuit Miniaturization
Stay updated, free articles. Join our Telegram channel
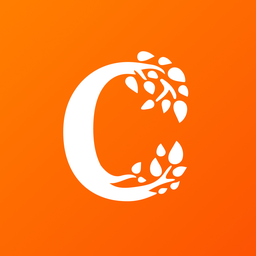
Full access? Get Clinical Tree
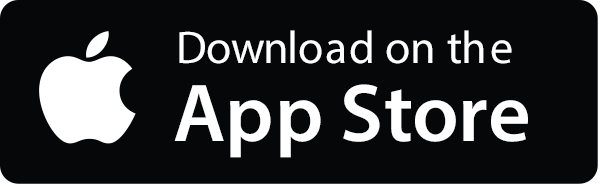
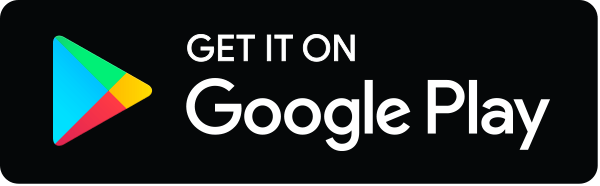