Introduction
Carbon dioxide (CO 2 ) is produced during aerobic cellular respiration and is a “waste product” produced by all aerobic life forms. Arterial CO 2 tension (arterial P co 2 ) represents the balance between CO 2 produced, the CO 2 eliminated, and in some cases inspired (either rebreathed or directly added to the breathing circuit) CO 2 . Inspired CO 2 (F ico 2 ) is usually negligible, while alterations in CO 2 production are an unusual—but possible—contributor to systemic CO 2 tension. As an example, CO 2 production increases in hypermetabolic states such as fever or thyrotoxicosis; however, these are generally offset by modest increases in alveolar ventilation, sufficient to normalize the arterial P co 2 . Therefore, for practical purposes, the most important determinant of arterial P co 2 is its rate of elimination.
Hypocapnia remains a common—and generally underappreciated—component of many disease states, including early mild asthma, high-altitude pulmonary edema, and acute lung injury. In addition, deliberate induction of hypocapnia remains a common, if controversial, practice in both adults and children with acute brain injury, as a strategy to control raised intracranial pressure. In contrast, hypercapnia has historically been avoided by clinicians, with high tidal volumes and minute ventilation employed to normalize arterial P co 2 , in an effort to keep it within a “normal physiologic” range.
Advances in current understanding of the biology of CO 2 have prompted the idea that hypocapnia or hypercapnia may play roles in the development of acute organ dysfunction or injury. In preclinical models, deliberate hypercapnia may protect against lung and systemic organ injury, beyond effects of reduced tidal volume. In addition, hypocapnia may exert independent harmful effects. Limited clinical data suggest potential beneficial effects of hypercapnia in patients exposed to high lung stretch. This chapter reviews the current clinical status of hypocapnia and hypercapnia in health and disease, discusses the insights gained from basic studies of CO 2 , identifies key unresolved concerns regarding hypocapnia and hypercapnia, and considers the potential clinical implications for the management of patients with lung disease.
Regulation of Arterial CO 2 Tension
In health, arterial P co 2 is tightly regulated via a feedback loop between CO 2 tension and alveolar ventilation. CO 2 has a fundamental role in regulating and controlling breathing, and hypercapnic acidosis is a potent ventilatory stimulant. Type I glomus cells in the carotid body near the bifurcation of the carotid artery and chemosensitive neurons in numerous brain stem regions respond to changes in pH and arterial P co 2 . During normal tidal respiration, the carotid chemoreceptors are critical for maintaining stable arterial P co 2 levels. The response time of the peripheral chemoreceptors to transient changes in arterial P co 2 is more rapid than that of the central neurons ; however, the central chemosensitive neurons exert a quantitatively larger contribution to stimulating ventilation in response to hypercapnia.
The effect of alterations in arterial P co 2 on ventilation is—at least in part—pH dependent, via the production of protons following its spontaneous and carbonic anhydrase–catalyzed combination with water to form carbonic acid, which in turn dissociates to form bicarbonate ( ) and H + ions. Changes in CO 2 and CO 2 /H + levels are sensed in special chemosensitive neurons in the carotid body and in several regions of the hindbrain, although it is not certain whether these cells detect pH, CO 2 ,
, or a pH gradient across the cell membrane. Nonetheless, the ventilatory response to hypercapnia is greater than that to metabolic acidosis, suggesting the existence of a CO 2 receptor. The peripheral chemoreceptors appear to respond primarily to alterations in arterial P o 2 and H + concentrations, and their function is to maintain arterial P o 2 and H + concentrations constant. The central chemoreceptors appear to respond primarily to alterations in CO 2 /H + levels and act to maintain the H + concentration of the cerebrospinal fluid constant. Any acute change of H + in these compartments is counteracted almost instantly by alterations in pulmonary ventilation and more slowly corrected by the kidney.
Causes of Altered Arterial CO 2
Arterial P co 2 is usually expressed in terms of a relationship among CO 2 production, CO 2 elimination, and rarely, a contribution from inspired CO 2 (F ico 2 is usually close to zero).

Accidental Hypercapnia or Hypocapnia
Accidental hypercapnia is reported most commonly in the operating room or the intensive care unit, and is due to errors associated with mechanical ventilation. The key errors are those that result in hypoventilation, such as circuit disconnection or inadequate minute ventilation. Alternatively, expired gas (which contains approximately 5% CO 2 ) can be rebreathed as a result of circuit malconnection or depletion of CO 2 absorbers.
Additional causes of acute hypercapnia include hypoventilation secondary to drug-induced respiratory depression, or severe airways obstruction such as status asthmaticus or massive aspiration (e.g., grain inhalation). Increased dead space (e.g., pulmonary embolism) will result in decreased alveolar ventilation and hypercapnia if the minute ventilation is fixed (e.g., the patient is paralyzed on mechanical ventilation), although in most cases, the patient will increase minute ventilation to maintain CO 2 in the normal or slightly low range. Chronic hypercapnia may result from progressive restrictive or obstructive lung diseases such as pulmonary fibrosis or chronic obstructive airways disease or obstructive sleep apnea. Increases in CO 2 production can result from hypermetabolic states such as fever, sepsis, malignant hyperthermia, thyroid crisis, or sometimes overfeeding. In addition, use of bicarbonate buffers (NaHCO 3 ) results in generation of excess CO 2 . Finally, significant increases in CO 2 production will directly increase arterial P co 2 if the alveolar ventilation is not increased; however, even a slight increase in alveolar ventilation will easily compensate for this.
In the setting of mechanical ventilation, accidental hypocapnia might be more common than accidental hypercapnia, especially in the absence of end-tidal CO 2 monitoring, such as outside the operating room. In one study, severe hypocapnia (end expired CO 2 < 30 mm Hg) was found in 70% of patients transferred by helicopter to a U.S. trauma center. In children, accidental hypocapnia readily develops during high-frequency ventilation or during extracorporeal support (e.g., extracorporeal membrane oxygenation [ECMO], cardiopulmonary bypass).
Permissive Hypercapnia
Permissive hypercapnia refers to the elevated arterial P co 2 that results from hypoventilation of mechanically ventilated patients that is aimed at reducing ventilator-associated lung injury (e.g., acute respiratory distress syndrome [ARDS], status asthmaticus).
Deliberate hypoventilation was initially described in 1984 by Darioli and Perret, wherein a series of 29 mechanically ventilated patients (34 episodes of ventilation) with status asthmaticus were managed with deliberate hypoventilation (resulting in severe hypercapnia) with the goal of reducing barotrauma. There were no fatalities in the series, which was very unusual at the time for intubated patients with status asthmaticus. The following year, Wung and colleagues described a series of 15 neonates with persistent pulmonary hypertension of the newborn. This case series was remarkable given that, up to then, vigorous hyperventilation (to achieve hypocapnia in an attempt to reduce the pulmonary vascular resistance) was considered a vital aspect of management; in such cases, death was common and chronic lung disease the rule. There were no deaths in the series of Wung and colleagues and only a single case of chronic lung disease.
Subsequently, the term “permissive hypercapnia” was coined by Hickling and associates in their seminal descriptions of improved survival in ARDS in which plateau pressures and tidal volumes were limited. In all these series, hypercapnia was tolerated (permissive); it was not specifically induced. Rather, the aim was to diminish barotrauma from excessive tidal volumes and airway pressure, and the hypercapnia was simply a result of this strategy. Nonetheless, because of these advances, clinicians became more accepting of hypercapnia in critically ill patients.
Deliberate Hypercapnia or Hypocapnia
Historically the administration of inspired CO 2 was used to hasten emergence from anesthesia; the idea was that stimulation of spontaneous ventilation enhanced clearance of inhaled volatile anesthetic gases (of course increasing minute ventilation using the mechanical ventilator would equally clear the volatile gases, but would result in profound hypocapnia and therefore prolonged apnea). Such administration of inhaled CO 2 at the end of anesthesia was originally termed de-etherization, and the practice was discontinued in the 1980s, largely because of concerns with inadvertent hypercapnia.
More recent clinical studies confirm that therapeutic hypercapnia hastens emergence from anesthesia, even with the more insoluble modern anesthetic agents, and capnography—not available in the 1980s—can help avoid excess hypercapnia. Such hypercapnic hyperpnea in spontaneously breathing patients can reduce by half the required recovery time from anaesthesia and could have benefits for postoperative cognitive function and operating room efficiency.
Therapeutic hypercapnia refers to the concept that elevated CO 2 may have specific benefits in critical illness, beyond benefits resulting from lessening barotrauma. If this concept proves to be clinically applicable, then “therapeutically” elevating arterial P co 2 might be beneficial in ARDS, in addition to tidal volume reduction. Whereas there is some preclinical evidence to suggest benefit for hypercapnia in specific experimental models, this approach has not been directly tested in critically ill patients.
In contrast, therapeutic hypocapnia is often used in the management of acute brain injury in both adults and children. Hypocapnia is induced to lower intracranial pressure (ICP) by decreasing the cerebral blood volume through cerebral artery vasoconstriction. Because elevated ICP is generally adverse and hypocapnia has traditionally been thought to be benign, hyperventilation has been widely practiced in patients with acute brain injury, even in the absence of elevated ICP. This reasoning led to the idea that more profound hyperventilation might be even better, and sometimes extremes of hypocapnia were advocated for acute brain injury, although more recently this concept has been discredited.
Transport of CO 2 in the Blood
The vast bulk of CO 2 is produced in the mitochondria, where cellular CO 2 concentrations are highest. The pathway for transportation, involving step-wise decreases in CO 2 partial pressure gradients, originates in the mitochondria and proceeds through the cytoplasm, cell membranes, capillaries, venules, larger veins, and ultimately into the mixed venous blood before elimination through the alveoli.
Transportation of CO 2 in the blood is accomplished via three different mechanisms with the exact proportions carried by each mechanism varying depending on whether it is arterial or venous blood. Dissolved CO 2 in plasma, reported as arterial P co 2 (i.e., partial pressure) accounts for only 5% to 10% of the total CO 2 transported in blood. Almost 90% of total CO 2 in the blood is converted to bicarbonate ions ( ), almost all catalyzed by carbonic anhydrase within the red blood cells. The remainder (5% to 10%) is transported as carbamino-hemoglobin, in which CO 2 is bound to terminal amino groups in hemoglobin (Hb) molecules. The usual amount of CO 2 in the arterial blood is 21.5 mmol per liter of blood, with slightly more (23.3 mmol/L) in venous blood. Overall, more than 80% of the CO 2 is carried within the red blood cells.
Oxygen-Induced Hypercapnia
CO 2 transport in the blood is altered by oxygen, leading to an elevated P co 2 ; this oxygen-induced hypercapnia is seen in patients with end-stage lung disease who inhale supplemental O 2 . The mechanism of oxygen-induced hypercapnia was formerly thought to be oxygen-induced inhibition of ventilatory drive in patients thought to be critically dependent on hypoxic ventilatory drive. In fact, minute ventilation is not diminished in such patients. The mechanism is now better understood as having three key components: the Haldane effect, impaired hypoxic pulmonary vasoconstriction, and inability to increase minute ventilation.
The Haldane effect is the term given to the phenomenon whereby increasing arterial P o 2 reduces the ability of the blood to store CO 2 (as Hb-bound, carbamino Hb; or as ), thereby increasing the CO 2 partial pressure. There are two elements to the Haldane effect. First, increased arterial P o 2 decreases formation of carbamino compounds; this reduces the quantity of CO 2 bound to Hb, thereby elevating the dissolved CO 2 (P co 2 ). Second, histidine is important for the buffering properties of Hb; it contains an imidazole group that, at physiologic pH, is an effective buffer of H + ions but is also an important molecular link between heme groups and the Hb chains. Elevated P o 2 results in greater quantities of O 2 bound to Hb, which causes allosteric modifications of the Hb confirmation. These conformational changes impact the heme-linked histidine and reduce its ability to buffer H + ion; with less H + buffering by Hb, there is more H + binding to
and release of stored CO 2 .
In patients with end-stage lung disease, hypoxic pulmonary vasoconstriction is an important mechanism to divert pulmonary artery blood from poorly ventilated regions (see Chapters 4 and 6 ). Increasing arterial P o 2 inhibits hypoxic pulmonary vasoconstriction, thus pulmonary artery blood containing CO 2 is diverted to less well-ventilated regions, and the efficiency of CO 2 excretion is impaired. Finally, while most patients would easily compensate for the increased P co 2 with minimal increases in minute ventilation, this is not possible in many patients with end-stage lung disease.
Molecular Effects of CO 2
Acid Base and Ionic Balance
CO 2 plays a key role in acid-base regulation, because the bicarbonate-CO 2 buffer system is the predominant buffer in the bloodstream. During metabolic acidosis, excess H + ions in the blood combine with to form the weak (carbonic) acid H 2 CO 3 . Hypercapnia generally results in acidosis, by combining with water to form carbonic acid, which in turn dissociates to form bicarbonate (
) and H + ions. The protons thus generated can react with titratable groups in certain amino acids, resulting in structural changes in many proteins and enzymes in cell membranes and cellular aqueous environments. Because acidosis suppresses most cellular functions, the body uses a number of strategies to defend its intracellular and extracellular pH within remarkably narrow limits. The intracellular acidosis produced by hypercapnia may be corrected within a few hours, as opposed to 1 to 2 days needed for renal compensation. This buffering is accomplished via active cell membrane ion transporters that extrude protons and exchange them for extracellular sodium.
CO 2 has effects on intracellular and extracellular ion concentrations. Following the onset of hypocapnia, there is rapid efflux of hydrogen ions; this compensatory mechanism is exhausted easily and, if hypocapnia persists, systemic alkalosis develops. The kidney effects a more efficient compensatory mechanism, reducing its hydrogen ion excretion and increasing bicarbonate loss, as well as decreasing excretion of ammonium. During (hypocapnic) alkalemia, movement of H + from intracellular to extracellular space is accompanied by an opposite movement of K + (and Na + ) into the cell, although the resulting hypokalemia is usually modest. In addition, phosphate moves into the cell because of increased cellular phosphorylation. During alkalemia, albumin releases bound H + , swapping it for Ca 2+ , and lowering the ionized calcium fraction, which may be severe.
Protein Function and Metabolic Effects
CO 2 molecules can bind directly with free amine groups in proteins to form carbamate residues. This binding of CO 2 also modifies protein structure and function and may account for some of the observed differences in the effects of acidosis caused by CO 2 compared to acidosis resulting from other causes. The Bohr effect, wherein increased arterial P co 2 results in a rightward shift of the hemoglobin-oxygen dissociation curve, resulting in a lowered affinity of Hb for O 2 and facilitation of oxygen unloading at the tissues, is a good example of this phenomenon.
One consequence of intracellular alkalosis is activation of glycolysis due to inhibition of the rate-limiting enzyme phosphofructokinase. A feedback system functions as a mechanism to generate H + ions to counter intracellular alkalosis, whereby lactic acid production is increased by alkalemia (and decreased by acidemia).
Respiratory System Effects
Pulmonary Vasculature
The effects of CO 2 on the pulmonary vasculature contrast with those in the systemic circulation. Hypercapnia can increase pulmonary vascular pressure by increasing both cardiac output and pulmonary vascular resistance ( Table 86-1 ).While hypercapnia increases pulmonary arterial pressure and pulmonary vascular resistance, this effect might not be as significant in preexisting pulmonary hypertension. Hypercapnia is a less potent pulmonary vasoconstrictor than hypoxia, and its more important effect may be in augmenting hypoxic vasoconstriction. While relatively little is understood about the cellular mechanism of CO 2 -induced pulmonary vasoconstriction, much of this effect appears to be catecholamine-mediated.
Organ System | Physiologic Effects | Pathophysiologic Consequences |
---|---|---|
Cardiovascular | Cardiac output and myocardial perfusion Increased cardiac output Direct reduction in cardiac contractility Counterbalanced by indirect sympathoadrenal effects (increased preload, heart rate, myocardial contractility) and decreased afterload (reduced vascular tone) Coronary vasodilation, mainly mediated by nitric oxide | Improved cardiac output and oxygen delivery to tissues |
Systemic oxygen delivery Rightward shift of hemoglobin-oxygen dissociation curve (Bohr effect) May acutely increase hematocrit | Improved oxygen delivery and unloading in tissues | |
Oxygen demand HCA reduces cellular respiration and oxygen consumption | Reduction in tissue O 2 demand | |
Respiratory | Control of respiration Changes in CO 2 /H + levels sensed in special chemosensitive neurons in the carotid body and in hindbrain | Oxygen-induced hypercapnia (see text for mechanism) |
Pulmonary vascular resistance Increased cardiac output and pulmonary vascular resistance Augments hypoxic pulmonary vasoconstriction | Acute hypercapnia can worsen pulmonary hypertension Enhances ventilation-perfusion matching | |
Airway resistance Direct dilation of small airways Indirect—vagally mediated—large airway constriction | Opposing actions of CO 2 may result in little net change in airway resistance Contribution to ventilation-perfusion matching (e.g., during pulmonary embolism) | |
Lung compliance Increased surfactant secretion Increased parenchymal compliance | Improved lung compliance | |
Gas exchange CO 2 modifies pulmonary vascular and airway tone to help match regional ventilation and perfusion | Improved matching of ventilation and perfusion Increased arterial oxygenation by this mechanism in both normal and injured lungs | |
Neurologic | Intracranial pressure Increased cerebral blood flow and volume | Increased intracranial pressure |
Cerebral oxygenation Increased cerebral blood flow Increased cerebral oxygenation | Increased cerebral oxygenation—potential benefit in ischemic states | |
Cerebral oxygen demand Decreases central nervous system O 2 demand | Improved cerebral oxygen supply-demand ratio | |
Inflammation and immunity | Neutrophil and macrophage function Inhibition of neutrophil and macrophage migration and adhesion Decreased secretion of important proinflammatory cytokines (e.g., TNF-α, IL-8, IL-6) Inhibits phagocytosis and intracellular killing of bacteria (by reduction of nicotinamide adenine dinucleotide phosphate-oxidase activity) | Reduced host response to injury Reduced proinflammatory response Potential increased susceptibility to prolonged sepsis |
Free radicals Decreased xanthine oxide free radical generation Decreased production of NO-derived radicals (NO 2 , NO 3 ) and may shift the balance from O 2 -derived toward N 2 -derived (i.e., nitration) reactions | Reduced free radical mediated injury Reduced microbicidal efficacy | |
Metabolic | Intracellular metabolism Intracellular acidosis causes inhibition of glycolysis due to inhibition of the rate-limiting enzyme phosphofructokinase Feedback system whereby lactic acid production is decreased by acidemia | Decreased metabolism—potentially beneficial in ischemic states Decreased metabolic demand |
Hypocapnic alkalosis produces pulmonary vasodilation, which is commonly used to reduce elevated pulmonary artery pressure, particularly in the setting of congenital heart disease and persistent pulmonary hypertension of the newborn ( Table 86-2 ).
Organ System | Physiologic Effects | Pathophysiologic Consequences |
---|---|---|
Cardiovascular | Cardiac output and myocardial perfusion Reduced cardiac output Increased systemic vascular resistance Hyperventilation additionally impairs venous return Decreased myocardial blood flow, independent of myocardial workload Increased myocardial oxygen extraction (as a result of hyperventilation) | Reduced cardiac output May worsen myocardial ischemic injury |
Systemic oxygen delivery Leftward shift of the hemoglobin-oxygen dissociation curve (increased affinity of hemoglobin for oxygen) Compensated to some extent by a rapid increase in lactate production and by an increase in the concentration of 2,3-diphosphoglycerate over a period of several hours Systemic arterial vasoconstriction decreases global and regional O 2 supply | Decreased oxygen delivery to tissues | |
Oxygen demand Increased cellular excitation and oxygen demand | Oxygen supply/demand imbalance | |
Respiratory | Control of respiration Changes in CO 2 /H + levels sensed in special chemosensitive neurons in the carotid body and in hindbrain | Cheyne-Stokes respiration and central sleep apnea are thought to be due to decreased cerebral chemosensitivity to CO 2 and left ventricular systolic dysfunction |
Pulmonary vascular resistance Hypocapnic alkalosis produces pulmonary vasodilation | Acute hypocapnia is used to alleviate pulmonary hypertension especially in setting of congenital heart disease and persistent pulmonary hypertension of the newborn Worsens ventilation-perfusion matching | |
Airway resistance Direct constriction of small airways | Contribution to ventilation-perfusion matching, which could be beneficial (e.g., during localized perfusion deficits such as pulmonary embolism), or harmful (e.g., during asthma) | |
Lung compliance Increased alveolar permeability, decreased compliance, and reduced surfactant production These effects can be ameliorated by normalizing alveolar CO 2, and in some cases prevented by elevated inspired CO 2 | Reduced lung compliance and worsened lung injury | |
Gas exchange Worsened bronchoconstriction Reduced collateral ventilation and impaired parenchymal lung compliance Attenuated hypoxic pulmonary vasoconstriction and increased intrapulmonary shunting | Worsens matching of ventilation and perfusion The overall effect can be a net decrease in arterial P o 2 Attenuation of hypoxic pulmonary vasoconstriction; can worsen intrapulmonary shunt and systemic oxygenation | |
Neurologic | Intracranial pressure (ICP) Reduced ICP via arteriolar vasoconstriction and decreased cerebral blood volume | Acute hypocapnia decreases ICP, which is potentially lifesaving where ICP is critically elevated |
Cerebral oxygenation Decreases cerebral blood flow Decreases systemic oxygenation | Decreases cerebral oxygenation—potentially harmful in ischemic states Cerebral vasoconstriction may reduce overall perfusion ; in focal ischemia, blood flow to hypoxic regions is selectively lowered and infarct size increased Termination of sustained hypocapnia can precipitate cerebral hyperemia and raised intracranial pressure | |
Cerebral oxygen demand Increased CNS O 2 demand through increasing neuronal excitability, through increased excitatory synaptic transmission, and by direct effect on the neuronal membrane itself | Worsens cerebral oxygen supply-demand ratio | |
Acid-Base | Initial rapid cellular efflux of hydrogen ions (which is exhausted easily) Followed by renal compensatory mechanism: reduced hydrogen ion excretion, increased bicarbonate loss, and decreased excretion of ammonium | Movement of H + from intracellular to extracellular space is accompanied by (1) an opposite movement of K + (and Na + ) into the cell (the resulting hypokalemia is usually modest ), (2) phosphate movement into the cell due to increased cellular phosphorylation, and (3) albumin release of bound H + , swapping it for Ca 2+ and lowering the ionized calcium fraction, which may be severe |
Metabolic | Intracellular alkalosis causes activation of glycolysis due to inhibition of the rate-limiting enzyme phosphofructokinase Feedback system whereby lactic acid production is increased by alkalemia | Increased tissue O 2 demand |
Airway Resistance and Lung Compliance
CO 2 can impact airway tone, with hypocapnia producing bronchoconstriction and hypercapnia causing bronchodilation in isolated airway preparations. Hypercapnia has been variably reported to either increase or decrease airway resistance. This paradox may be explained by direct dilation of small airways and indirect—vagally mediated—large airway constriction. Indeed, in spontaneously breathing unanesthetized subjects, the CO 2 -related changes of respiratory mechanics are entirely caused by upper airway resistance. In mechanically ventilated subjects, hypercapnia may decrease airway resistance, although opposing actions of CO 2 may result in little net change in airway resistance.
Parenchymal lung compliance increases in response to hypercapnic acidosis, arising from active relaxation in response to increased alveolar CO 2 or due to increased surfactant secretion or more effective surface tension–lowering properties under acidic conditions.
Gas Exchange
In the normal lung, CO 2 modifies pulmonary vascular and airway tone. Increasing alveolar P co 2 causes bronchodilation, while increasing pulmonary arterial P co 2 increases pulmonary vascular resistance. The physiologic role of these contrasting effects of CO 2 on bronchial versus pulmonary arterial smooth muscle tone is to facilitate matching of regional ventilation and perfusion. For example, pulmonary artery occlusion results in regional hypocapnia, which results in bronchoconstriction, thereby directing airflow away from these unperfused alveoli. By this same mechanism, CO 2 administration improves matching of ventilation and perfusion and increases arterial oxygenation in both normal and injured lungs.
Alveolar CO 2 can alter distribution of ventilation among acini and larger lung units, as well as augment global ventilation-perfusion matching, which leads to improved gas exchange. A dose-response relationship exists between F ico 2 and arterial P o 2. However, in ARDS, permissive hypercapnia (produced via lowering tidal volume) is associated with increased shunt, although this is likely due to atelectasis from low tidal volumes.
Severe hypocapnia produces bronchoconstriction, reduces collateral ventilation, and impairs parenchymal lung compliance. Hypocapnia also attenuates hypoxic pulmonary vasoconstriction and increases intrapulmonary shunting. The overall effect can be a net decrease in arterial P o 2 .
Hypocapnic alkalosis impairs pulmonary capillary permeability in the rodent isolated lung. In contrast, hypercapnia reduces alveolar fluid clearance in healthy isolated perfused lungs by endocytotic withdrawal of Na + /K + -ATPase from the basolateral membrane of alveolar epithelial cells. Whether this is the case in vivo or in injured lungs remains to be determined.
Diaphragmatic Function
The effects of hypercapnia on the diaphragm are complex and incompletely understood. Hypercapnia has been demonstrated to impair diaphragmatic function in preclinical studies in which alveolar ventilation was not controlled. Rats exposed to prolonged environmental hypercapnia (7.5% CO 2 for 6 weeks) demonstrate significantly depressed diaphragmatic muscle function, as well as altered diaphragmatic composition with increased slow-twitch and decreased fast-twitch fibers. In fact, even 15 minutes of exposure to 7% inspired CO 2 may transiently and reversibly impair neuromuscular function. In contrast, in studies in which ventilation is controlled and CO 2 increases moderately, hypercapnia may protect the diaphragm from injury. Thus it remains unclear whether any deleterious effects of hypercapnia on the diaphragm are due to direct effects of CO 2 or result from diaphragmatic fatigue from the induced increase in ventilation.
Central Nervous System Effects
Cerebral Blood Flow
The change in cerebral blood flow (CBF) during variations of arterial P co 2 depends on several factors, including baseline CBF, cerebral perfusion pressure, and the presence of anesthesia. Across a broad range of conditions, most studies report an increase in global CBF of 1 to 2 mL • 100 g −1 • min −1 for each 1 mm Hg increase in arterial P co 2 ; reducing arterial P co 2 to 20 to 25 mm Hg decreases the global CBF by 40% to 50%, with minimal effect from further arterial P co 2 reduction. Increasing the arterial P co 2 to 80 mm Hg produces a maximal increase in CBF of 100% to 200% in anesthetized animals, but in the awake state, arterial P co 2 of 80 mm Hg can increase CBF by up to sixfold; in such cases, one half of the increase in CBF results from release of endogenous catecholamines and activation of neuronal metabolism. Thus, in awake subjects, severe hypercapnia may increase the flow by two mechanisms: a direct effect of CO 2 on cerebral blood vessels and an indirect effect increasing neuronal metabolic rate and catecholamine release.
However, these effects are not sustained: with hypocapnia for up to 4 hours, CBF recovers to within 10% of baseline due to reduced cerebrospinal fluid and extracellular and due to correction toward a normal extracellular pH.
The extent to which CO 2 modulates CBF varies depending on baseline CBF, which is not homogeneous across the brain. Areas of the brain that receive more blood flow have a steeper flow response to changes in arterial P co 2 . In patients with traumatic brain injury, there is a 3% change in CBF per mm Hg change in arterial P co 2 , but because the response depends on baseline flow, it is reduced in patients with lower CBF. The regional differences are significant. In the cerebral cortex of the anesthetized cat, the baseline CBF is 86 mL•100 g −1 •min −1 , and a 1 mm Hg change in arterial P co 2 changed CBF by 1.7 mL. In contrast, the spinal cord has a blood flow of 46 mL•100 g −1 •min −1 , and each 1 mm Hg change in arterial P co 2 changed CBF by 0.9 mL. Similar findings have been reported in rabbits and humans.
Hypocapnia and Cerebral Vasoconstriction
The mechanisms by which arterial CO 2 tension modulates cerebral vasoactivity depend on the size and type of blood vessel. The effects on cerebral vascular tone are mediated via changes in pH rather than CO 2 per se. The diameter of cerebral arterioles responds only to changes in pH and not to buffered changes in CO 2 tension. In addition, the effect of CO 2 on cerebrovascular tone is largely confined to the cerebral arterial vasculature, with little effect on associated veins or capillaries.
Hypercapnic acidosis induces precapillary dilation of cerebral arterioles, and whereas all cerebral arteries show some responsiveness to altered CO 2 tension, the larger arteries are less sensitive while the small pial arterioles are most responsive. Changes in these arterioles are not detected using noninvasive technologies, such as transcranial Doppler, which focuses on the larger vessels.
Whereas direct and indirect mechanisms mediate the effects of CO 2 on CBF, cerebral vascular tone is altered by CO 2 only via a direct effect on the arteriole wall and not by alterations in catecholamine release or sympathetic tone. The vascular endothelium and smooth muscle layer play a central role. Several lines of evidence suggest that endothelial release of nitric oxide (NO) in response to alterations in P co 2 is a key mechanism. Administration of an exogenous NO donor reduces basal cerebrovascular resistance and blunts the cerebral vasoconstrictor effect of hypocapnia in humans. Damage to cerebrovascular endothelium is associated with impaired CO 2 cerebrovascular reactivity, which parallels impaired release of NO from the endothelium. Inhibition of NO synthase reduces hypercapnia induced cerebral vasodilation. Specific inhibition of the neuronal isoform of NO synthase reduces hypercapnia induced vasodilation in the parietal cortex and retinal circulation.
In contrast, in adult rodents, damage to the endothelium in vivo does not alter the response of the cerebral arteries to hypercapnia, suggesting alternative pathways. CO 2 ultimately regulates vascular tone by modulating intracellular calcium concentration and sensitivity. Alkalosis induces increases in concentrations of intracellular calcium in the vascular smooth muscle, increasing the vascular tone. In addition, NO activates guanylate cyclase in vascular smooth muscle, increasing the cyclic guanosine monophosphate concentration, which in turn phosphorylates calcium channels.
Elevation of extracellular calcium prevents acidosis-induced dilation of cerebral arterioles, suggesting that reduced vascular smooth muscle calcium reentry may mediate in part the acidosis-reduced vascular tone. In addition, opening of potassium channels during acidosis allows egress of potassium, hyperpolarizing the cell, closing voltage-gated calcium channels, and reducing vascular tone by reducing calcium entry.
Cerebral Perfusion—Flow Versus Volume
The primary aim of inducing hypocapnia in the setting of raised ICP is to reduce cerebral blood volume ( Fig. 86-1 ). However, the effect of CO 2 on cerebrovascular tone is largely confined to the cerebral arteries ; thus the effect of changing arterial P co 2 on CBF is substantially larger than its effect on cerebral blood volume ( Fig. 86-2 ). Fortune and colleagues demonstrated that hypocapnia in volunteers decreased cerebral blood volume by 7% while reducing CBF by greater than 30%. Because low CBF is common in the first 24 hours after traumatic brain injury, there is particular concern for inducing ischemia by hyperventilation. In summary, the cost of hypocapnia in terms of reduced cerebral perfusion may outweigh any benefit in terms of reducing intracranial volume. Even so, hypocapnia remains in widespread use in the management of patients with acute brain injury ( Fig. 86-3 ).



Cerebral Oxygenation
In humans, when arterial P co 2 is reduced to 20 to 25 mm Hg, CBF is reduced to 20 to 25 mL•100 g −1 •min −1 , but does not reach lower levels even during extreme hypocapnia in anesthetized (arterial P co 2 10 mm Hg) or nonanesthetized humans (arterial P co 2 16 mm Hg). In nonanesthetized, normothermic humans and primates, the earliest signs and symptoms of cerebral ischemia, such as confusion, inability to follow commands, focal neurologic deficits, and slowing of the electric activity of the brain, measured by an electroencephalogram, are seen at global CBF levels of 20 to 30 mL•100 g −1 •min −1 ; however, CBF must be reduced to less than 10 mL•100 g −1 •min −1 to cause neuronal cell death.
Hyperventilation can reduce CBF to ischemic levels; in humans and animals, reducing the arterial P co 2 to 20 to 25 mm Hg slows the electroencephalogram and impairs mental function, both of which suggest mild cerebral ischemia. Severe hypocapnia also increases central nervous system (CNS) lactate, consistent with impaired oxidative metabolism, and cortical P o 2 is reduced during hypocapnia. Indeed, severe hypocapnia (10 mm Hg arterial P co 2 ) can reduce cortical P o 2 despite a plateau low level of CBF, indicating that further hypocapnia additionally increases local O 2 consumption or inhibits local delivery. Marked alkalosis also shifts the oxyhemoglobin dissociation curve to the left, which can enhance uptake in the lung, but limits oxygen delivery to systemic organs, including the brain.
Hypocapnia increases neuronal excitability via a direct effect on the neuronal membrane, which increases cerebral glucose utilization, leads to neuronal glucose depletion, and increases anaerobic metabolism. Also, alkalosis, especially respiratory alkalosis, inhibits the usual negative feedback by which low pH limits production of endogenous organic acids (e.g., lactate) during ischemia. This may prevent cellular metabolic down-regulation in the setting of diminished nutrient supply, a potentially protective mechanism.
Overall, most studies indicate that, in the normal brain, hypocapnia can reduce CBF to the point of cerebral ischemia but not to levels of CBF that cause rapid neuronal death. If hypocapnia causes cerebral ischemia in the normal brain, it is likely mild and not associated with gross disturbances of brain oxidative metabolism. However, the long-term effect of hyperventilation on the normal brain is not known. Thus, in aggregate, hypocapnia may cause brain ischemia by increasing cerebral oxygen demand as well as decreasing cerebral oxygen supply. These concerns limit the utility of hypocapnia to the setting of life-threatening increases in ICP, where it can be lifesaving by allowing time for definitive intervention.
Cardiovascular System Effects
Systemic Circulation
Hypercapnic acidosis directly reduces contractility of cardiac and vascular smooth muscle. This is counterbalanced by the hypercapnia-mediated sympathoadrenal effects of increased preload and heart rate, increased myocardial contractility, and decreased afterload, leading to a net increase in cardiac output. Hypercapnia results in a complex interaction of altered cardiac output, hypoxic pulmonary vasoconstriction, and intrapulmonary shunt to produce a net increase in arterial P o 2 . Because hypercapnia generally elevates cardiac output, global O 2 delivery is increased. Hypercapnic acidosis causes coronary vasodilation, mainly mediated by NO. Hypercapnia and acidosis shift the hemoglobin-oxygen dissociation curve rightward, reducing the oxygen affinity of Hb, and hypercapnic acidosis may acutely elevate hematocrit by a mechanism that may involve sympathetically mediated autotransfusion, further increasing tissue oxygen delivery. Acidosis may reduce cellular respiration and oxygen consumption, which may further benefit a supply and demand imbalance. In addition, hypercapnic acidosis increases O 2 tension in both subcutaneous tissues and the intestinal wall.
Hypocapnia and alkalosis, on the other hand, increase the affinity of Hb for oxygen (leftward displacement of the dissociation curve) and reduce microcirculatory blood flow, further impairing local oxygen delivery. This is compensated to some extent by a rapid increase in lactate production and by an increase in the concentration of 2,3-diphosphoglycerate, which, over a period of several hours, may have an opposite effect on the dissociation curve.
Hypocapnia depresses myocardial blood flow, independent of myocardial workload. In patients with coronary artery disease, mild hyperventilation slightly increases systemic vascular resistance and reduces cardiac index. In addition, hyperventilation increases myocardial oxygen extraction. More pronounced levels of hypocapnia reduce cardiac output, and indirect effects of intrathoracic pressure associated with passive hyperventilation may additionally impair venous return and further depress cardiac output.
Splanchnic Effects of Altered CO 2
Hypercapnia improves regional—including mesenteric—blood flow and enhances peripheral microcirculatory flow, thereby increasing organ oxygen delivery and splanchnic tissue oxygenation. Hypercapnia preserves tissue oxygenation of the gastrointestinal mucosa in uninjured animals, and it reverses decrements in gastric mucosal oxygenation during hemorrhagic shock and following captopril administration.
The effects of CO 2 on the splanchnic organs are less well understood. CO 2 and acid-base status modulate splanchnic organ cellular function. Acidosis protects hepatocytes from anoxic or chemical hypoxia-induced cell death, while restoration of normal pH abolishes this protection. This effect may represent a protective adaptation against hypoxic and ischemic stress. In the kidney, acidosis protects isolated renal cortical tubules from anoxia-induced injury. Hypercapnic acidosis, particularly in the setting of concomitant hypoxia, may produce marked sympathetic activation, which can lead to intense renal vasoconstriction and tubular sodium reabsorption, causing depressed glomerular filtration and increased fluid retention.
Inflammation and Repair
Mediator Production
Hypercapnia and acidosis inhibit cytokine signaling in immune effector cells. Acidosis reduces secretion of important proinflammatory cytokines (e.g., TNF-α, IL-8, IL-6) from polymorphonuclear leukocytes (PMNs) and macrophages ; especially important may be the production of IL-8 by PMNs, which is pivotal in mediating the acute inflammatory response to infection. However, the inhibition of PNM IL-8 secretion is blocked by pretreatment with acetazolamide, a drug that lessens the impact of P co 2 on neutrophil intracellular pH.
CO 2 -induced inhibition of cytokines can be sustained. Indeed, peritoneal macrophages had reduced TNF-α production up to 3 days after peritoneal insufflation of CO 2 (as used during laparoscopic surgery), a phenomenon that may result from inhibition of nuclear factor-kappa B (NF-κB), a regulator of the transcription of mediators in inflammation, injury, and repair.
Complement Activation
Innate immunity requires the complement system in order to target pathogens for phagocytosis or lysis; complement is activated by acidosis. Acid activation of C5 combines with C6, forming a lytic complex, C5b6a via the classic activation pathway. The alternative complement pathway is also activated by acidosis with increased activity of C3 convertase, in turn increasing complement binding to—and lysis of—erythrocytes. C3 (and C5) is activated with H + from hypercapnic or lactic acidosis, as well as with hydrochloric acid, and is due to the acidosis per se.
Free Radical Generation
Hypercapnic acidosis reduces free radical tissue damage in brain and lung, as well as following lung ischemia-reperfusion. It lessens production of NO-derived radicals (NO 2 , NO 3 ) following ventilator and endotoxin-induced injury, and may shift the balance from O 2 -derived toward N 2 -derived (i.e., nitration) reactions. This effect may be beneficial where greater injury is associated with oxidative versus nitration reactions. However, it is not clear whether hypercapnic acidosis reduces in vivo tissue oxidative damage beyond its impact on neutrophils.
Innate Immune Response
Neutrophils, macrophages, and circulating monocytes are major elements in the innate immune response, and are especially important in responses to bacterial infection. They are directly activated by bacteria, bacterial products, or inflammatory molecules such as complement. Neutrophils rapidly localize to areas of infection or inflammation, migrating from the bloodstream, and rapidly phagocytosing bacteria on contact. Phagocytosis is rapidly followed by bacterial lysis and destruction because the phagosomes fuse with toxic granules containing powerful enzymes such as elastases, proteases, nicotinamide adenine dinucleotide phosphate-oxidase (generates superoxide and hydrogen peroxide) and myeloperoxide (generates hypochlorous acid). Migration of PMNs is key and impairment of migration to infection sites worsens outcome in sepsis. Circulating monocytes (and their macrophage counterparts in tissues) coordinate lymphocyte activation in sepsis by presenting bacterial antigen and secreting chemokines, and macrophages are activated by bacteria or by molecules such as endotoxin or complement components. Finally, monocytes and macrophages phagocytose and kill pathogens by similar mechanisms as—but at a slower rate than—neutrophils.
Hypercapnic acidosis inhibits neutrophil and macrophage function, and this inhibition can be sustained. For example, ex vivo inhibition of peritoneal macrophages lasts up to 3 days. The mechanisms are unclear but hypercapnic inhibition of macrophage TNF-α production requires more than transient (∼30 min) exposure, happens despite normal TNF-α mRNA levels, and persists long after ambient CO 2 has normalized. Hypercapnia or acidosis may impact these processes in other ways, including alteration of intracellular pH, reduction of neutrophil recruitment or migration, inhibition of phagocyte oxidizing function, impairment of cytokine signaling among effector cells, or potentiating neutrophil death.
Phagocyte Migration, Chemotaxis, and Adhesion
Neutrophil migration is key to immune defense, and depends on processes such as cytoskeleton remodeling, membrane recycling (endocytosis, exocytosis), integrin-mediated focal adhesion attachment and reattachment, and regulation of cell volume. Many of these processes are pH dependent, and hypercapnic acidosis may therefore reduce neutrophil and macrophage recruitment to a septic focus. Acidosis impairs neutrophil chemotaxis and migration, and hypercapnic acidosis reduces neutrophil expression of chemokines, selectins, and intercellular adhesion molecules, which mediate the neutrophil-endothelial interactions required for migration out of blood vessels. Finally, hypercapnic acidosis reduces lung neutrophil migration following in vivo endotoxin.
Phagocyte Microbial Killing
Pathogens are internalized by neutrophils and macrophages within phagosomes, which merge with endosomes and lysosomes, and are lysed by powerful enzymes. This process requires bacterial phagocytosis and an intact “respiratory burst,” which produces the reactive oxygen species ( , H 2 O 2 , HClO) within the endosomes and lysosomes needed for bacterial killing. Acidosis—both hypercapnic and metabolic—impairs neutrophil and macrophage phagocytosis; specifically, failure to recover intracellular pH (pHi) after acidification impedes ROS production and killing of phagocytosed bacteria in macrophages, and metabolic or hypercapnic acidosis inhibits neutrophil phagocytosis. In contrast, moderate alterations of ambient pH have little effect on neutrophil phagocytosis, but markedly reduce subsequent bacterial lysis. Finally, incubation in organic acid inhibits macrophage phagocytosis.
One of the enzymes largely responsible for the production of these oxygen-derived radicals, nicotinamide adenine dinucleotide phosphate-oxidase, is markedly pH sensitive. For example, macrophage production decreases linearly with reduction in pHi (below 6.8), and hypercapnic and metabolic acidosis inhibits oxidant production in neutrophils that are nonstimulated or stimulated with a variety of chemical and microbial stimuli. Although marked reduction of extracellular pH to 6.5 may activate neutrophils (i.e., increasing H 2 O 2 and integrin CD18) this may reflect experimental use of strong HCl.
Mechanism of Phagocyte Inhibition
Regulation of pHi is key to cell function; in general, acidosis reduces enzyme activity and protein function, particularly when pHi is less than 6.8. Because CO 2 is diffusible, hypercapnia can rapidly lower pHi and thereby inhibit neutrophil and macrophage function. Two key systems regulate pHi in immune cells: the Na/H exchanger (NHE) and the plasmalemmal V-type H ATPase. These enzymes maintain pHi in the physiologic range (6.8 to 7.3), necessary for immune responses, as well as for other key functions including proliferation, differentiation, apoptosis, migration, cytoskeletal organization, and maintenance of cell volume.
Neutrophil pHi decreases following stimulation and activation and then reverts toward normal if extracellular pH is physiologic. This is important because PMNs that are unable to regulate cytosolic pH demonstrate impaired migration and apoptosis. However, extracellular hypercapnic acidosis rapidly lowers neutrophil pHi, and activated neutrophils from foci of inflammation are unable to maintain pHi following an acid challenge. Therefore persistent “acid loading,” as with ongoing permissive (or other) hypercapnia, could overwhelm the capacity of PMNs to maintain pHi and normal cell homeostasis and function. This may be especially so with PMNs that have already been activated.
Neutrophil Death
Neutrophils have a short life span (<48 hours after release into the circulation) that is terminated by apoptosis, a physiologic fate following phagocyte activity. In contrast, necrosis of neutrophils after phagocytosis is a maladaptive response because microbe killing and digestion may be incomplete, and in contrast to apoptosis, enzyme release causes tissue destruction. Lower extracellular pH—as with hypercapnic acidosis—may delay neutrophil apoptosis and extend PMN life span. However, hypercapnic acidosis also lowers pHi, which may increase neutrophil propensity to undergo necrosis rather than apoptosis. Thus the net effect of hypercapnic acidosis on neutrophil fate—whether beneficial or deleterious—remains unclear.
Adaptive Immune Response
Adaptive immunity is especially important in many cancers, which characteristically involve poor vascularization, tissue hypoxia, and acidosis, a setting wherein acidosis may impair host responses to tumor cells, resulting in tumor growth and metastases. However, the impact of acidosis is complex. For example, acidosis inhibits cytotoxicity of activated killer lymphocytes and natural killer cells, reduces lysis of tumor cells by cytotoxic T lymphocytes, and inhibits IL-2 stimulated lymphocyte proliferation. In contrast, IL-2 stimulated lymphocyte motility is enhanced by acidosis, as is the antigen presenting capacity of dendritic cells. The net effect of these contrasting actions of acidosis is unclear. However, hypercapnic acidosis does enhance murine tumor spread, raising concerns about hypercapnia-induced suppression of cell-mediated immunity.
Bacterial Proliferation
Hypercapnia and acidosis exert distinct effects on bacterial proliferation. For example, optimal anaerobic growth of Escherichia coli is seen at a P co 2 of approximately 0.05 atmospheres (similar to the usual gut E. coli environment); it is not inhibited at a P co 2 of approximately 0.2 atmospheres but is inhibited at greater than 0.6 atmospheres. Indeed, E. coli colony counts were halved at a CO 2 of approximately 350 atmospheres, a massive dose. Although the mechanisms are unclear, such inhibition is mediated directly by CO 2 , and the effects are broadly similar among microbes, with yeasts being more resistant and gram-negative organisms being more susceptible.
Although important, the levels of CO 2 in these studies are far higher than encountered in humans. In fact, clinically relevant levels of metabolic acidosis can enhance bacterial proliferation, as has been observed when mechanically stretched lung epithelial cells can develop lactic acidosis that augmented E. coli growth. Such acidosis-induced E. coli proliferation has been demonstrated due to H-ions (not CO 2 ) and is abolished by local buffering.
While many bacteria ( E. coli, Proteus mirabilis, Serratia rubidaea, Klebsiella pneumoniae, Enterococcus faecalis, and Pseudomonas aeruginosa ) isolated from patients with ventilator-associated pneumonia grew better in acidified media, at least one important pathogen (i.e., methicillin-resistant Staphylococcus aureus ) grew optimally at alkaline pH. In summary, while bacterial growth can be inhibited by extremely high levels of CO 2 , it can be enhanced by clinically relevant levels of acidosis; thus the net impact of hypercapnic acidosis on bacterial growth is a concern.
Effects of CO 2 on Repair and Resolution
Restoration of Epithelial Integrity
Elevated CO 2 directly inhibits alveolar epithelial plasma membrane resealing, and decreases repair of alveolar epithelial wounds, both important mechanisms contributing to repair following injury to the lung. Hypercapnic acidosis exerts a direct pH-dependent inhibition of lung epithelial cell membrane healing following ventilation-induced injury, a repair mechanism that depends on translocating lipids to the injured membrane. In contrast, the effect of elevated CO 2 on pulmonary epithelial wound repair appears dependent on the hypercapnia rather than the acidosis per se, and appears to be mediated via effects on cell migration. CO 2 (pH-independent) induction of microRNA-183 (miR-183), albeit with high P co 2 levels (120 mm Hg), may lead to mitochondrial dysfunction, which in turn impairs cell proliferation and survival.
Lung Permeability and Fluid Clearance
Lung water accumulation and clearance are important contributors to injury and repair, respectively. Hypercapnic acidosis directly reduces, while hypocapnic alkalosis worsens, ischemia-reperfusion–induced pulmonary capillary permeability. In contrast, hypercapnia reduces alveolar fluid clearance in healthy isolated perfused lungs. Hypercapnia appears to activate extracellular signal-regulated kinase, which in turn activates adenosine monophosphate–activated protein kinase, leading to endocytotic withdrawal of Na + ,K + -ATPase from the basolateral membrane of alveolar epithelial cells.
Cellular Sensing and Gene Activation
Mechanisms of Cellular Sensing
As discussed in the section on regulation of arterial CO 2 , cellular sensing of CO 2 is best characterized in central and peripheral nerve cells in relation to control of breathing. However, it remains unclear whether these chemosensitive neurons detect alterations in CO 2 directly or via changes in H + concentrations. In addition, (but not CO 2 or H + ) directly activates adenylate cyclase, increasing cyclic adenosine monophosphate and activating protein kinase A; this in turn results in opening of L-type Ca 2+ channels, permitting Ca 2+ influx. Such changes may mediate responses of putative (but as yet unidentified) CO 2 receptors. In addition, increased CO 2 (independent of pH) activates L-type calcium channels in the glomus cells of the carotid nucleus, also resulting in calcium influx.
Gene Activation
An important downstream consequence of cell sensing is gene activation. Key insights into CO 2 -induced gene activation have been gained by gene array studies in murine lung tissue, nematode (Caenorhabditis elegans), and the fruit fly (Drosophila melanogaster) following exposure to high concentrations of CO 2 .
Neonatal mice exposed to atmospheric hypercapnia demonstrated increased expression of lung genes involved in regulating cell adhesion, growth and signal transduction, and suppression of genes involved in innate immunity. Hypercapnia also suppressed lipopolysaccharide-induced cytokine production (and phagocytosis) by murine macrophages, possibly explained by concomitant suppression of NF-κB and consistent with impaired NF-κB signaling induced by hypercapnia in mouse embryonic fibroblasts and other mammalian cell types.
CO 2 exposure also caused altered gene expression in model organisms C. elegans and D. melanogaster, where such transcription regulation has been largely attributed to inhibition of the NF-κB family of transcription factors. For example, hypercapnia-impaired innate immune responses in D. melanogaster increased susceptibility to bacterial infection and were associated with suppression of Relish (an NF-κB homolog) and its associated genes.
Thus CO 2 causes common gene expression patterns that are conserved across diverse species. Key features include suppression of genes associated with innate immunity and inhibition of NF-κB signaling ( Fig. 86-4 ). NF-κB is sequestered in the cytosol by inhibitory molecules (IκBs), and inflammatory stimuli activate upstream signaling cascades, causing phosphorylation and degradation of IκB; this liberates NF-κB, permitting its translocation to the nucleus where it activates expression of genes involved in innate immunity and inflammation. CO 2 directly inhibits the NF-κB pathway, likely through CO 2 -induced nuclear localization of the IKKα subunit, thereby preventing degradation of IκB. The protective effects of hypercapnia in mechanical stretch–induced injury in vitro and in ventilation-induced lung injury in vivo appeared to be mediated via inhibition of the NF-κB pathway.

Pulmonary Pathophysiology
Preclinical Models
Hypercapnic acidosis is protective in several in vivo and ex vivo lung injury models, including lung injury caused by endotoxin, pulmonary, and mesenteric ischemia-reperfusion, in addition to ventilator-induced lung injury (see Fig. 86-4 ). In E. coli pneumonia, hypercapnia attenuates histologic injury by a neutrophil-independent mechanism, and it attenuates lung injury caused by short-term and more prolonged systemic polymicrobial sepsis. In contrast to acute pulmonary hypertension, chronic exposure to hypercapnia causes reversal of hypoxia-induced pulmonary hypertension in adult and neonatal rats, and protects against parenchymal and vascular injury in neonatal lungs.
In contrast to protective effects, hypercapnia has several adverse effects on immunity and repair. While protective in acute and more established lung sepsis, hypercapnia over a longer period (48 hours) is associated with increased bacterial proliferation and more marked lung injury. Furthermore, hypercapnia induced by lowered respiratory rate and tidal volume exacerbated endotoxin-induced lung injury and increased neutrophil adhesion and adhesion molecule expression. Finally, as discussed earlier, important aspects of injury resolution may also be impaired by hypercapnia, such as epithelial wound repair —likely via inhibition of NF-κB —and clearance of alveolar edema.
Hypocapnia has long been recognized as associated with adverse lung effects and was thought to be pathogenic in ARDS. Hypocapnia may contribute to lung injury in two ways. First, high tidal volume that induces hypocapnia can separately cause ventilator-induced lung injury. Second, hypocapnia may directly injure the lung in the absence of ventilator-induced injury and can increase permeability, decrease compliance, inhibit surfactant, and potentiate inflammation. These effects can be ameliorated by normalizing alveolar CO 2 , and in some cases the effects can be prevented by elevated inspired CO 2 . Finally, alveolar hypocapnia attenuates hypoxic pulmonary vasoconstriction, worsening intrapulmonary shunt, and systemic oxygenation.
Acute Respiratory Distress Syndrome
Trials of low tidal volume ventilation provide little direct insight into any independent role of CO 2 because patients have not been randomized to hypercapnia per se; in fact, in the large ARDS Network trial comparing target tidal volumes of 6 versus 12 mL/kg, there was only a mean 5 mm Hg greater arterial P co 2 in the lower tidal volume arm. Nonetheless, using the database from this large trial, multivariable analysis suggested that moderate levels of hypercapnic acidosis (pH 7.15 to 7.35; arterial P co 2 45 to 65 mm Hg) significantly lowered the odds ratio for death in the 12 mL/kg (but not in the 6 mL/kg) group, suggesting that permissive hypercapnia may limit ventilator-induced lung injury. In addition, a smaller study by Amato and colleagues reported reduction in mortality from a combination of lower tidal volume and higher positive end-expiratory pressure (resulting in substantially greater arterial P co 2 ) on survival in ARDS. This study and the retrospective studies on permissive hypercapnia demonstrate a link, but not a cause-and-effect relationship, between higher arterial P co 2 and improved outcome.
Asthma
Among common causes of respiratory failure, status asthmaticus is associated with the highest levels of hypercapnia (arterial P co 2 often greater than 100 mm Hg, pH often approaching 7) ; this is usually transient and well tolerated, and survival is the rule. In the first substantial series of patients with severe acute asthma requiring mechanical ventilation, institution of controlled hypoventilation (i.e., permissive hypercapnia), complications, and mortality were considerably lower than previously reported. Although such findings have been confirmed repeatedly, it is not possible to determine whether hypercapnia provided any benefit beyond minimization of barotrauma due to the lower tidal volumes.
Chronic Obstructive Pulmonary Disease
Hypercapnia in chronic obstructive pulmonary disease is associated with poor prognosis, although any prognostic impact of hypercapnia is confounded by the influence of other underlying factors, including alveolar loss, hypoxemia, and airway obstruction. However, in patients receiving long-term domiciliary O 2 , higher arterial P co 2 may be associated with lower mortality ; this would suggest a distinction between progressive hypercapnia due to terminal respiratory failure versus adaptive hypercapnia due to respiratory center recalibration and tolerance of higher arterial P co 2 , thereby lowering respiratory drive and lessening respiratory work. Such an “adaptive” mechanism would cause a progressive decline in arterial P o 2 due to progressively decreasing minute ventilation, but this could be addressed by supplemental oxygen therapy.
Neonatal and Pediatric Respiratory Failure
Hypercapnia appears to be safe and is associated with some benefits in the setting of neonatal and pediatric respiratory failure. Neonatal respiratory distress syndrome is a disorder of surfactant production in the preterm newborn and results in parenchymal stiffness and alveolar collapse. In this setting, ventilation strategies involving permissive hypercapnia (45 to 55 mm Hg) was demonstrated in a randomized clinical trial to facilitate weaning from mechanical ventilation, although there was no clear effect on chronic lung disease or survival in this small study ( Fig. 86-5 ). Data also exist supporting the use of permissive hypercapnia in other pediatric respiratory pathologies, such as congenital diaphragmatic hernia, pulmonary hypertension of the newborn, and congenital heart disease.

Central Nervous System Pathophysiology
Hypocapnia is widely used in the management of acute brain injury, and may be lifesaving when ICP is critically elevated, but the use of hypocapnia outside this specific setting can produce neuronal ischemia and injury, resulting in harm. Hypercapnia is usually avoided in the setting of acute brain injury because it increases CBF and volume, which may cause or exacerbate intracranial hypertension. Even so, hypercapnia may have protective effects in the brain in specific circumstances.
Preclinical Models
Hypocapnia has been demonstrated to exert potentially harmful effects in acute brain injury. Hypocapnia worsens ischemic brain injury, diminishes neuronal energy status (i.e., glucose utilization, phosphate reserves), and worsens CNS functional and histologic outcomes following experimental cardiac arrest in dogs. Severe hypocapnia increases N -methyl- d -aspartate receptor-mediated neurotoxicity in the newborn and may impair neuronal membrane function through increased incorporation of choline into membrane phospholipids.
Through increasing O 2 demand and decreasing supply, hypocapnia can adversely shift the global CNS O 2 supply-demand balance. Cerebral vasoconstriction may reduce overall perfusion, and in focal ischemia, blood flow to the hypoxic regions is selectively lowered and infarct size increased. Hypocapnia also increases CNS O 2 demand by increasing neuronal excitability, increasing excitatory synaptic transmission, and affecting the neuronal membrane itself.
Termination of sustained hypocapnia can precipitate cerebral hyperemia and raised ICP. During sustained hypocapnia, brain extracellular fluid becomes depleted of , thereby reducing buffering capacity; thus any subsequent increase in arterial P co 2 —because it is less buffered—will result in an exaggerated increase in CBF. This has been experimentally verified in rabbits and goats.
In contrast, hypercapnia may exert direct protective effects in the injured brain. Hypercapnic acidosis decreases disruption of the blood-brain barrier in hypertensive crisis and attenuates hypoxic-ischemic and hypoxia/reoxygenation brain injury. Hypercapnic acidosis is more effective than comparable degrees of metabolic acidosis in prevention of lipid peroxidation in cortical homogenates.
Traumatic Brain Injury
Hypocapnia is commonly used in the management of patients with traumatic brain injury despite there being no evidence to show that it improves outcome. On the contrary, a randomized controlled trial of prolonged hyperventilation in traumatic brain injury demonstrated that it worsened outcome. Patients with severe traumatic brain injury were randomized to normal (arterial P co 2 35 mm Hg) versus prophylactic hyperventilation (arterial P co 2 25 mm Hg) targets; among the less severely injured patients (Glasgow Coma Scale motor score 4-5), fewer in the hyperventilation group had a favorable outcome at 3, 6, and 12 months than did those managed with normocapnia. Important insights were gained from this study: there was greater ICP variability and higher mean ICP levels after 60 hours of hyperventilation. Thus hyperventilation was at best ineffective in terms of its primary rationale (i.e., reduction of ICP); in fact, it worsened ICP. Finally, the adverse effects of hypocapnia seemed less serious in a third study group who received hyperventilation plus buffering (with tromethamine); however, these patients may have been less severely injured.
Neonatal Brain Injury
Hypocapnia (either deliberate or accidental) is common in neonatal practice. Unfortunately it appears to be particularly injurious to the premature human brain, especially in neonatal white matter injury. Hypocapnia is a key risk factor for several specific lesions, including periventricular leukomalacia, causing significant neonatal mortality and long-term neurodevelopmental deficits ; pontosubicular necrosis, a pattern of acute brain injury seen in premature infants ; and the pathogenesis of cerebral palsy.
The mechanisms predisposing premature neonates to injury from hypocapnia include vulnerable regions due to poorly developed vasculature ; reduced defenses because of antioxidant depletion by excitatory amino acids ; lipopolysaccharide and inflammatory mediators potentiating white matter destruction.
Preterm infants exposed to severe (arterial P co 2 < 15 mm Hg) hypocapnia, even if only for a brief period, may develop considerable long-term neurologic abnormalities compared with matched, nonexposed controls. Risk factors for hypocapnia in small infants include hyperventilation, high-frequency ventilation, or ECMO. Indeed, hypocapnia in infants prior to the initiation of ECMO is associated with an increased incidence of sensorineural hearing loss by school age. Finally, abrupt termination of hyperventilation results in reactive hyperemia, which may cause intracranial hemorrhage, particularly in premature infants.
Acute Stroke
Hyperventilation has traditionally been used as a therapy in patients with acute stroke for two reasons. First, hypocapnia was thought to shunt blood preferentially to ischemic areas by selective vasoconstriction in normal autoregulated brain regions; this was called the inverse steal phenomenon. Second, hypocapnia was considered to correct regional acidosis adjacent to the ischemic zone, in order to minimize the extension of the infarct. However, the inverse steal phenomenon is no longer accepted ; in fact, hypocapnic alkalosis has been associated with poor prognosis in patients with acute cerebrovascular accidents. Cheyne-Stokes respiration and central sleep apnea, which can be observed after stroke, are thought to be due to decreased cerebral chemosensitivity to CO 2 in the setting of significant cerebral infarction and left ventricular systolic dysfunction.
Neuropsychological Impact
Marked intraoperative hypocapnia—even briefly—can cause detectable neuropsychological impairment for up to 48 hours after general anesthesia ; older patients are more susceptible with greater impairment after exposure to low CO 2 levels. The impairment includes slowed reaction times, as well as higher functions including attention, learning, and personality changes. More severe hypocapnia (arterial P co 2 of approximately 15 mm Hg) lowers psychomotor performance in healthy volunteers ; in contrast, elevated arterial P co 2 during anesthesia is associated with improved neuropsychological performance. Hypocapnia is also associated with adverse neurologic outcome following cardiopulmonary bypass, although in this setting there are multiple additional contributors.
Whereas adverse neuropsychological effects of transient hypocapnia appear to be reversible, this is not the case following prolonged exposure. Impairment following exposure at extremes of altitude can be long-standing; the deficits are better correlated with the levels of hypocapnia versus the degree of hypoxemia, consistent with the idea that individuals who can develop the lowest arterial P co 2 levels—required to maximize alveolar O 2 content and minimize hypoxemia—are most at risk. Thus a paradox exists: climbers with greater levels of physical fitness, that is, who can develop the greatest minute ventilation, may be the most vulnerable to CNS sequelae. It is likely that profound CNS alkalosis is the basis for the deficit: intense cerebral vasoconstriction and left-shift of the hemoglobin-oxygen dissociation curve markedly diminish O 2 delivery, negating any advantage of increased oxygen loading in the lung, while alkalosis concomitantly increases neuronal cell excitability and local O 2 consumption. Such alkalosis is ameliorated by acetazolamide pretreatment. Finally, it is likely that induced hypocapnia (e.g., with mechanical ventilation) may result in more severe CNS compromise in those with preexisting neurologic deficit.
In contrast, as discussed earlier, hypercapnia appears to speed restoration of consciousness and neurocognitive function following anesthesia. The deleterious effects of hypocapnia on neurocognitive function may therefore be reversed by CO 2 administration.
Cerebral Ischemia
The impact of hypocapnia on cerebral ischemia is most apparent in neuropsychological studies and in studies of cerebral perfusion or oxygenation in traumatic brain injury, cardiac arrest and resuscitation, and neonatal brain injury and stroke.
An initial response in traumatic brain injury is reduced cerebral perfusion, which is worsened by hypocapnia, and even moderate levels (<34 mm Hg) can reduce overall CBF and increase the proportion of injured brain that is inadequately perfused. This is of particular concern because regional flow deficits can be critical in common conditions including brain contusions, underlying hematomas, and diffuse injury. The responsiveness of the cerebral vasculature to CO 2 is increased following brain trauma, increasing the likelihood of ischemia and consistent with the worsening of outcome that is associated with prophylactic hyperventilation in such patients. Hypocapnia has also been shown to induce critical cerebral ischemia in pediatric traumatic brain injury.
Neurologic deficit is perhaps the most important complication of cardiac arrest. Following reestablishment of spontaneous circulation, the cerebral O 2 supply and demand balance is altered, with CBF at approximately 50% of normal but with cerebral O 2 consumption increased above prearrest levels. In this setting, hypocapnia causes cerebral ischemia and may therefore contribute to adverse outcome.
Premature infants are especially susceptible to a variety of brain injury syndromes, and hypocapnia contributes to several of these, including periventricular leukomalacia. In terms of outcome, prior exposure of premature infants to hypocapnia increases the risk of neurologic deficits, including sensorineural hearing loss.
Finally, outcome following acute stroke appears to be worsened by hypocapnia, which is consistent with experimental data demonstrating that hypocapnia increases the extent of ischemic injury.
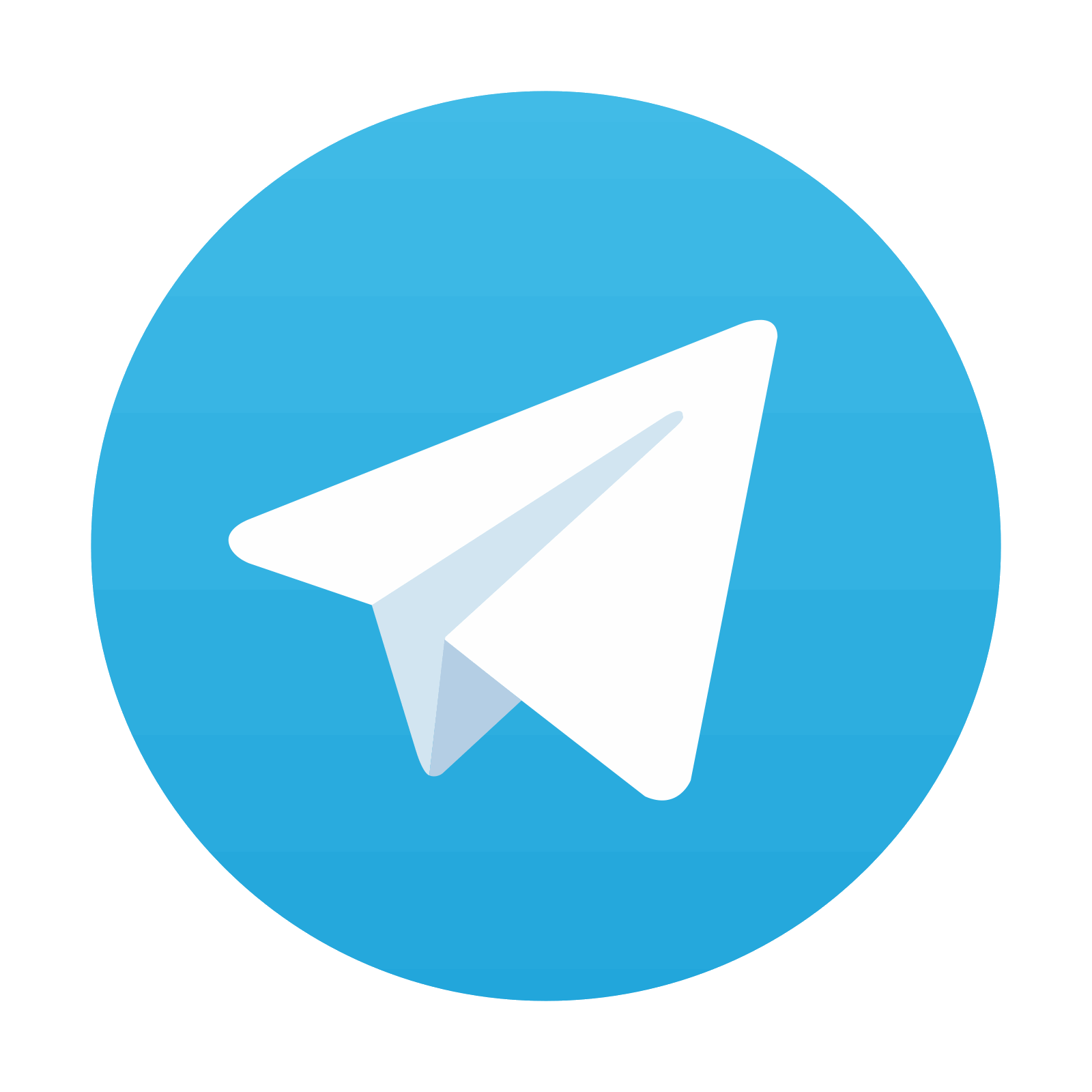
Stay updated, free articles. Join our Telegram channel
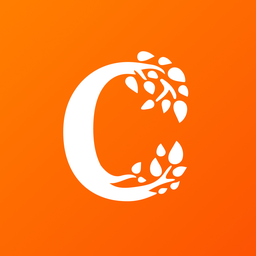
Full access? Get Clinical Tree
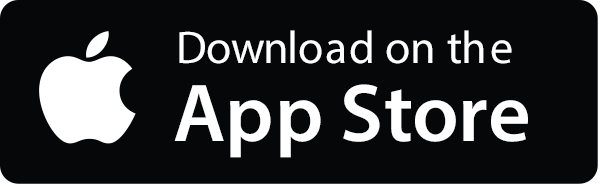
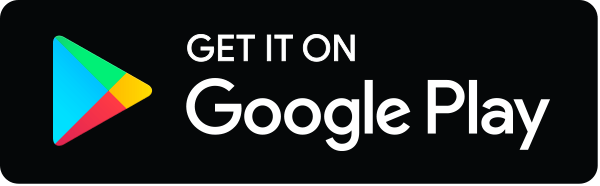