Introduction
Nearly 100 million people live at altitudes greater than 2500 m, primarily in the Rocky Mountains of North America, the Andes Mountains of South America, the Ethiopian Highlands of East Africa, and the Himalaya Mountains of South-Central Asia. These people have developed the ability to live and reproduce at elevations as high as 5000 m, but in some cases, develop chronic medical problems attributable to their high-altitude residence. In addition to long-term residents, many lowlanders venture to high altitude for work and recreation. These more acute exposures also pose the hazards of acute altitude illnesses or altitude-related exacerbations of preexisting diseases. With growing awareness of such risks, persons are seeking advice on traveling to these regions. It is, therefore, imperative that the counseling physician be able to provide guidance about minimizing risks and managing problems.
This chapter reviews the normal adaptive processes that take place in response to high altitude, specifically the compensatory changes in the transport of oxygen from the air to the tissues in the setting of decreased barometric pressure. Acute and chronic adaptation are discussed, and factors determining the limits of adaptation and performance at high altitude are explored. Also reviewed are the consequences of maladaptation—the acute and chronic illnesses of high altitude—and strategies for managing these situations are discussed. Finally, an approach to the traveler with underlying medical problems who is planning a trip to high altitude is presented.
Adaptation to High Altitude
The partial pressure of oxygen (P o 2 ) diminishes as oxygen moves from air to the tissues. Ventilation, regional matching of ventilation and blood flow, diffusion of oxygen from air to the blood, transport within the circulation, diffusion of oxygen from blood into the tissue, and metabolism in the mitochondria are all links in this oxygen transport chain. As altitude increases, the barometric pressure declines ( Table 77-1 ); as a result, P o 2 at every step in this chain is lower than it would be at sea level. Compensatory processes take place at each step in the chain at different rates to raise P o 2 and maintain adequate oxygen delivery, thereby facilitating tolerance of the hypoxic environment.
Altitude (meters) | Altitude (feet) | Barometric Pressure (mm Hg) | Inspired P o 2 (mm Hg) |
---|---|---|---|
0 | 0 | 760 | 159 |
1000 | 3280 | 674 | 141 |
2000 | 6560 | 596 | 125 |
3000 | 9840 | 526 | 110 |
4000 | 13,120 | 463 | 97 |
5000 | 16,400 | 405 | 85 |
6000 | 19,680 | 354 | 79 |
8000 | 26,240 | 268 | 56 |
8848 | 29,028 | 253 | 43 |
* Values except 8848 m, are taken for mid-latitude (45° N). There is greater variation at higher latitudes.
The process of adaptation is vitally important to survival at high altitude. Whereas a person who makes a sudden ascent from sea level to the summit of Mt. Everest (8848 m) by helicopter, for example, would become unconscious within minutes and die soon thereafter, a climber using an appropriately slow ascent rate can complete the climb without obvious effects because of the body’s adaption to the hypoxic environment. Since the latter half of the nineteenth century, researchers have investigated the full spectrum of these adaptive responses, creating a vast literature that has been reviewed extensively by Swenson and Bartsch and West and colleagues. A summary of the key features of the adaptive responses to high altitude follows.
Pulmonary Adaptation
Control of Ventilation
Acute Response.
Upon acute exposure to hypoxia, alveolar ventilation increases. This response, the hypoxic ventilatory response (HVR), minimizes the drop in alveolar P o 2 ( Fig. 77-1 ). Individuals vary in this response, which subsequently plays an important role in adaptation, maladaptation, and performance at high altitude. Large increases in ventilation are generally not seen until the arterial P o 2 falls below 60 mm Hg and, at any given altitude, ventilation continues to increase over weeks, in a process called ventilatory acclimatization . At extreme elevations, the increase in minute ventilation can be dramatic, representing a large metabolic cost. Data obtained from several individuals just below the summit of Mt. Everest (at 8400 m, barometric pressure approximately 272 mm Hg) revealed an average arterial P co 2 of 13 mm Hg. As a result, despite an inspired P o 2 of only 47 mm Hg, the mean arterial P o 2 was maintained at roughly 30 mm Hg.

The HVR is mediated by peripheral chemoreceptors in the carotid body. Neural discharge from the whole carotid sinus nerve is related hyperbolically to decreasing arterial P o 2 , and surgical ablation of carotid body input (e.g., carotid endarterectomy or bilateral carotid body resection for intractable dyspnea) results in loss of the ventilatory response to acute hypoxia.
In response to a relatively constant hypoxic stimulus, ventilation increases over time ( Fig. 77-2 ). With initial exposure and the subsequent acute rise in minute ventilation, arterial P co 2 at the medullary and peripheral chemosensors decreases and depresses the ventilatory response. Subsequently, over days, ventilation increases and continues to rise for months. The classic explanation for this ongoing increase was that the slower renal compensation for the respiratory alkalosis permitted near full realization of the hypoxic stimulus to ventilation. However, blood pH reveals that hypocapnic alkalemia persists as ventilation increases, suggesting that other mechanisms beyond renal compensation, which is completed within 3 to 4 days, must be responsible for this change. It was proposed that a relative acidosis in the cerebrospinal fluid (CSF) and at medullary chemosensors triggers the increased ventilation but lumbar CSF remains alkaline during high-altitude (3100 m) sojourns of up to 3 weeks, while during deacclimatization, hyperventilation persists even when arterial P co 2 and blood and CSF pH have returned toward normal. The pH of brain interstitial and intracellular fluid has also failed to show changes that could account for the increase in ventilation. These results suggest that some mediator other than hydrogen ion sustains the hyperventilation during a high-altitude sojourn. One possible mediator may be erythropoietin signaling in the brain via erythropoietin receptors. Another factor that would promote progressive hyperventilation is an increase over time in carotid body sensitivity to hypoxia.



Intermittent Hypoxic Exposure.
The sustained increase in ventilation generally pertains to exposures lasting days to weeks. Shorter intermittent exposures also affect ventilatory responses to both oxygen and carbon dioxide. Studies have focused on the “dose” and frequency of the hypoxic stimulus necessary to induce a physiologic response. For example, 8 hours of exposure to isocapnic and poikilocapnic (i.e., varying P co 2 ) hypoxia (end-tidal P o 2 = 55 mm Hg) increases the chemosensitivity to carbon dioxide compared to controls, while a 1 hr/day exposure to a simulated 4500-m altitude was associated with increased HVR, ventilatory equivalents ( ), and arterial S o 2 during postexposure hypoxia.
Chronic Ventilatory Adaptation.
Long-term high-altitude residents have lower ventilation and higher arterial P co 2 than acclimatized lowlanders at the same elevation and have blunted ventilatory responses to acute hypoxia. The blunted response arises in conjunction with carotid body hypertrophy and may be an adaptive phenomenon in which the disadvantage of the lower arterial P o 2 is offset by the decreased work of breathing associated with lower ventilation.
Pulmonary Function and Mechanics
On ascent, lung function changes in several ways. Vital capacity decreases in the first 24 hours and remains depressed at high altitude. Proposed mechanisms for a lower vital capacity include increased interstitial fluid volume, resulting in airway narrowing, gas trapping, and delayed emptying of some lung units, pulmonary vascular engorgement, decreased respiratory muscle strength, and reduction in lung compliance by hypocapnia. In addition, peak expiratory flow rates are increased and airway resistance is reduced. The lower airway resistance is presumably due to decreased air density, which counteracts any narrowing of airways due to hypoxia and hypocapnia.
In contrast, vital capacity is increased in high-altitude residents compared to that in nonnative residents, with the magnitude of the increase dependent on the length of stay at high altitude. Those born and raised at high altitude develop larger vital capacities than those who move to high altitude later in life.
Gas Exchange
Ventilation and Perfusion Matching.
The increase in ventilation during acute hypoxia is matched by increased cardiac output and pulmonary perfusion. Additionally, alveolar hypoxia triggers hypoxic pulmonary vasoconstriction (HPV), which increases pulmonary vascular resistance. These changes cause redistribution of blood flow to areas of the lung that are usually less well perfused at sea level and may, thereby, improve matching and optimize gas exchange.
Diffusion.
Several factors limit diffusion of oxygen from the alveolus to blood at high altitude. Decreasing barometric pressure lowers alveolar P o 2 , which, in turn, decreases the pressure gradient for diffusion such that at altitudes above 2500 m even the estimated pulmonary capillary transit time at rest of 0.75 second may not be adequate for end-capillary equilibration of oxygen; diffusion limitation is exacerbated during exercise due to the shortened transit time of blood across the pulmonary capillary and the greater fall in mixed venous P o 2 . Because the low arterial P o 2 lies on the steep portion of the oxygen-hemoglobin dissociation curve, a small decrease in P o 2 will result in a proportionately greater drop in pulmonary capillary oxygen content than at sea level. West and Wagner modeled these factors for the summit of Mt. Everest (P B ~ 250 mm Hg) and found that alveolar-capillary equilibration of oxygen is far from complete ( Fig. 77-3 ).


With long-term residence at high altitude, the diffusing capacity of the lung increases, although the mechanism for this increase remains unclear. When compared to sojourners, high-altitude residents have a lower (A − a)P o 2 difference, increased diffusing capacity, and despite having lower ventilation, a higher arterial P o 2 at rest and during exercise.
Cardiovascular Adaptation
Cardiac Response
With acute hypoxia and decreased arterial oxygen content, cardiac output increases in an effort to maintain oxygen delivery so that, for any given work rate, cardiac output is higher than at sea level. After about 1 week, the relationship between cardiac output and work rate returns to that seen at sea level. The increase in cardiac output is explained largely by an increase in heart rate rather than a change in stroke volume. In fact, stroke volume actually declines upon initial exposure to high altitude and remains lower than sea-level values despite acclimatization. The lower stroke volume is not due to depressed myocardial function, according to studies that reveal evidence of preserved cardiac contractility in hypoxia ; instead, it is likely due to diminished preload from a reduction in plasma volume. Systemic blood pressure increases in sojourners upon acute exposure to high altitude, likely due to increased sympathetic activity, but decreases over time. In fact, in as few as 1 to 2 years after moving to high altitude, many people have lower systemic pressure, likely secondary to a vasodilatory effect of hypoxia on systemic arterial smooth muscle.
Hemodynamic Response
At high altitude, low alveolar P o 2 triggers vasoconstriction in the pulmonary vasculature, a response known as hypoxic pulmonary vasoconstriction, or HPV. Pulmonary artery pressure increases minimally until the alveolar P o 2 drops below 70 mm Hg, at which point a more marked rise ensues ( Fig. 77-4 ). There is, however, substantial interindividual variability in this response.


Chronic hypoxic exposure results in even greater increases in pulmonary vascular resistance and pulmonary hypertension due to pulmonary vasoconstriction, hyperviscosity from polycythemia, and extensive vascular remodeling due to smooth muscle hypertrophy and collagen proliferation. The precise time course of these changes is not clear. In a 40-day simulated ascent of Mt. Everest (Operation Everest II), Groves and coworkers exposed individuals to progressively lower inspired oxygen tensions and demonstrated an increase in pulmonary vascular resistance of more than threefold. Interestingly, after the 40 days, breathing 100% oxygen did not return the pulmonary vascular resistance to the sea-level baseline. This result suggests that the vasculature can remodel in just a few weeks. Depending on the severity of changes, particularly the degree of intimal fibrosis, reversal of pulmonary hypertension may require months or years at low altitude.
Hematologic Adaptation
Erythropoietic Response
Hemoglobin concentration increases within 1 to 2 days of ascent and continues to rise for a number of weeks. The initial rise is due to hemoconcentration from a diuresis; further changes are due to increased red blood cell production, a result of hypoxia-mediated release of erythropoietin by the kidneys. Serum erythropoietin levels increase within 24 to 48 hours of ascent, and then decline over 3 weeks as acclimatization progresses. Both red blood cell volume and total blood volume increase. The erythropoietic response ceases on descent and hematocrit returns to sea-level values in approximately 3 weeks.
Although hematocrit generally increases with altitude, there is interindividual variability in the responses of both sojourners and highlanders. Similar variability is seen among different highland populations, which may be genetically determined : Andean natives demonstrate greater erythropoiesis than either Himalayan or Ethiopian highlanders. Whether greater erythropoiesis in Andean natives is secondary to genetic factors, environmental exposures, such as high levels of cobalt in and around mining regions, or an up-regulation of the erythropoietic response to the renal sensing of hypoxia is not clear.
While increasing hemoglobin concentration can augment arterial oxygen content, it may also impair oxygen delivery, in that, as the hematocrit approaches 60%, viscosity increases sufficiently to impair cardiac output and limit perfusion of the microvasculature.
Oxygen-Hemoglobin Affinity
Oxygen transport by blood is also influenced by the oxygen affinity of hemoglobin, as defined by the shape and position of the oxygen-hemoglobin dissociation curve. An important feature of the oxygen-hemoglobin relationship is the manner in which the dissociation curve steepens as arterial P o 2 falls below 60 mm Hg. As a result, with ascent to high altitude, arterial P o 2 falls into a range in which the oxygen content of hemoglobin drops precipitously with only small decreases in P o 2 .
To mitigate the potentially large impact on oxygen delivery, adaptations take place in the oxygen-hemoglobin relationship. For example, with initial exposure to high altitude and the resultant respiratory alkalosis, the dissociation curve shifts to the left. This shift enhances oxygen loading in the lungs and increases arterial oxygen content but comes at the expense of potentially decreased oxygen off-loading in the tissues. Over time, the curve shifts back to the right in response to increased 2,3-diphosphoglycerate concentrations and renal compensation for the respiratory alkalosis and improves oxygen off-loading in the tissues.
Coagulation
Hemostasis has not been shown to change in a consistent manner, with conflicting results regarding the effect of high altitude on platelet counts, bleeding times, and markers of thrombin and fibrin formation. Increased fibrinogen levels and prolonged clot lysis times in patients with high-altitude pulmonary edema (HAPE) have been taken as evidence that thrombosis and platelet aggregation may contribute to this disease. Bartsch and associates, however, performed careful longitudinal studies while subjects were developing HAPE and concluded that platelet and coagulation changes had no causative role. Thromboembolic risk at altitude may be linked to the underlying coagulopathy, but more work is necessary on this question.
Tissue Adaptation
At the final stage of oxygen delivery, oxygen moves from plasma to the site of oxidative phosphorylation in the mitochondria. Driving pressures of 10 mm Hg may be needed for movement from capillary plasma to the cytoplasm while driving pressures of only 1 to 2 mm Hg are necessary for diffusion from cytoplasm to the mitochondria. At sufficiently high altitudes, the capillary P o 2 may fall to levels at which diffusion from plasma into the mitochondria is impaired. Structural and biochemical responses (e.g., diminished muscle fiber size that reduces diffusion distance from blood to mitochondria, increased myoglobin concentration, and increased levels or activity of enzymes involved in oxidative metabolism) help overcome this problem. Much of this structural and metabolic adaptation may be driven by gene transcription via hypoxia inducible factors 1 and 2, which are known to regulate many hundreds of genes involved in tolerance to hypoxia, such as erythropoietin, vascular endothelial growth factor, nitric oxide synthase, and numerous enzymes in intermediary metabolism. Finally, recent evidence indicates that many cells express cytoglobins, heme proteins with oxygen-binding and transport characteristics similar to myoglobin, which may be up-regulated in hypoxia and play a role in adaptive responses.
Central Nervous System Adaptation
Cerebral Blood Flow
On acute exposure, hypoxia triggers an increase in cerebral blood flow that is, in part, offset by hypocapnic-mediated cerebral vasoconstriction; the net result is a modest increase in cerebral blood flow, which appears to be equally distributed between white and gray matter. The acute cerebrovascular response may be biphasic in nature with an initial decrease in cerebral blood flow due to acute hypocapnia followed by a subsequent increase triggered by arterial hypoxemia. After 4 to 5 days, cerebral blood flow decreases but is still 13% greater than the sea-level value. This increase in cerebral blood flow helps maintain oxygen delivery when arterial oxygen content is at its lowest until other adaptive mechanisms take effect and raise arterial oxygen content.
Cerebral Function
As one ascends, motor, sensory, and complex cognitive abilities are progressively impaired. New tasks are learned with difficulty at 3048 m, and simulated altitudes of 6100 m result in a decrement in sensory, perceptual, and motor performance. Short-term memory is impaired in climbers ascending without supplemental oxygen to 8500 m or higher. Even acute, modest decreases in arterial S o 2 to 85% impair mental concentration and fine motor coordination; a further decrease to 75% results in poor judgment, irritability, and decreased muscle function.
It is difficult to predict which individuals will experience neurologic dysfunction at high altitude, but combined data from a field study on Mt. Everest and a chamber study simulating an ascent of Mt. Everest (Operation Everest II), demonstrated that individuals with a high HVR and higher arterial S o 2 had more pronounced neuropsychometric dysfunction. One explanation for these findings is that those with a more brisk HVR might have greater hypocapnic cerebral vasoconstriction and, consequently, lower cerebral oxygen delivery.
A recent study in which brain magnetic resonance imaging (MRI) was performed on climbers who had ascended to summits between 5895 and 8848 m demonstrated evidence of subcortical lesions suggestive of small infarctions. The study did not correlate these findings with symptoms or signs of neurologic dysfunction and did not include follow-up studies to determine whether these lesions resolved, but the results raise the question as to whether high-altitude exposure causes permanent cerebral injury and whether the observed dysfunction at high altitude persists over time. Existing evidence suggests that the transient deterioration in learning, memory, and expression of verbal material return to baseline within 1 year but that fine motor coordination problems may persist for longer periods of time.
Cellular Adaptive Mechanisms
As with cytoglobin up-regulation in peripheral tissues, intracerebral expression of neuroglobin, another protein related to hemoglobin and myoglobin, is increased during hypoxic stress and may play a role in limiting neuronal dysfunction or injury.
Fluid Homeostasis and Renal Function
Upon ascent to high altitude, there is diuresis and natriuresis and, as with the HVR, these peripheral chemoreceptor-mediated responses vary almost 10-fold between individuals over the first 24 to 48 hours at altitude. Following acclimatization, further gains in elevation may lead to additional diuresis and natriuresis. The mechanism underlying this observed diuresis and natriuresis is not clear, but it does not appear to result from changes in renin, angiotensin, aldosterone, or atrial natriuretic peptide levels. In acute hypoxia, urinary protein excretion increases twofold to threefold, and with high-altitude illness, urinary protein excretion increases further.
Changes in Common Sea-Level Activities at High-altitude
Sleep
Difficulty sleeping is a common problem at high altitude. In studies examining pharmacologic prophylaxis for acute mountain sickness (AMS), for example, more than 70% of people receiving placebo reported poor-quality sleep. In addition to subjective impressions of sleep impairment, there is objective evidence of poor sleep, with increased arousals, changes in sleep architecture, and an increased incidence of periodic breathing ( ). In addition, it is during sleep that both sojourners and natives experience the most severe hypoxemia, because steep, transient drops in oxygen saturation often accompany apneic periods.
The mechanisms underlying periodic breathing at high altitude have been reviewed elsewhere. The consensus is that sleep eliminates most cortical influences on respiration, leaving hypoxemia via carotid chemoreceptors as the prominent drive. The effect of hypoxia-induced changes in P co 2 on hypoxic drive may set up oscillations of ventilation in a poorly damped system. At moderate altitudes (<3000 m), this periodic breathing diminishes over several days as ventilatory acclimatization proceeds, but at higher altitudes periodic breathing persists for long periods of time.
Periodic breathing during sleep at high altitude can be eliminated by breathing supplemental oxygen ( Fig. 77-5 ) and can be reduced through the use of respiratory stimulants such as acetazolamide. A review of trials of acetazolamide in AMS prophylaxis demonstrates that sleep was also improved in the acetazolamide groups compared to the placebo groups. More recently, the benzodiazepine class drug temazepam has also been shown to reduce the incidence of periodic breathing and improve measures of sleep quality without a negative effect on next-day reaction times or maintenance of wakefulness. Gamma aminobutyric acid receptor agents, including zolpidem and zaleplon, can also improve sleep quality and sleep architecture, but there is no evidence that either agent decreases periodic breathing.

Exercise
Exercise at high altitude is different in many important respects from exercise at sea level.
Maximal Work
With acute and prolonged hypoxic exposures, maximal oxygen consumption ( ) and work capacity are decreased relative to sea-level values.
decreases about 10% per 1000 m of altitude gain so that, at approximately 0.5 atm,
is about half of its sea-level value and, at 0.33 atm (as at the summit of Mt. Everest),
is about 23% of that at sea level. Because maximum oxygen uptake is decreased at altitude, maximum minute ventilation and heart rate at peak exercise are also reduced compared to their sea-level values ( Fig. 77-6 ).




Ventilation
Because of the decrease in air density and the lower amount of oxygen, greater ventilation is required to achieve the same oxygen uptake at high altitude. As a result, the ventilatory response to exercise is augmented at altitude compared to sea level. For example, with a sojourn to 6300 m, the ventilation for a given metabolic rate (the ventilatory equivalent, ) is almost four times as great as at sea level. The increase is a function of both the altitude attained and the strength of the individual’s HVR. The increase in ventilation comes at a price, however. At extreme altitude, the oxygen cost of breathing during exercise may be as high as 40% of the overall metabolic rate and may result in allocation of cardiac output to respiratory muscles that could otherwise be dedicated to muscles of locomotion. Extreme dyspnea with exercise may affect the intensity and duration of exercise; with rest, dyspnea typically resolves rapidly.
Gas Exchange
Unlike at sea level, where arterial oxygen saturation remains constant with increasing workloads in most normal individuals, it decreases with increasing workloads following either short ( Fig. 77-7 ) or long duration high-altitude exposures. Alveolar hypoxia decreases the driving gradient for diffusion while pulmonary capillary transit time is shortened as a result of the increase in cardiac output, thereby creating a true diffusion limitation (see Fig. 77-3 ). mismatching may also contribute to the observed gas exchange abnormalities ; nonuniform hypoxic pulmonary vascular response may lead to interstitial pulmonary fluid accumulation, which, in turn, creates
heterogeneity and impairs gas exchange.

Cardiovascular Response
For any given submaximal work rate, cardiac output and heart rate are higher during exercise at altitude compared to sea level. With acclimatization, cardiac output at submaximal workloads returns to sea-level values while the heart rate remains elevated. However, maximal exercise capacity and peak heart rate and cardiac output at altitude are reduced relative to sea-level values. With acclimatization, maximal exercise capacity is not restored to sea-level values over time and maximum cardiac output, heart rate, and stroke volume all remain decreased.
Pulmonary artery pressures reach high levels during exercise at high altitudes. Whether these high pressures limit exercise capacity at altitude is not clear. On the one hand, because cardiac output is maintained at levels appropriate for the work rates attained, the increase in pulmonary vascular resistance in healthy sojourners to high altitude would not appear to limit exercise. However, in a field study of acclimatized individuals, administration of the phosphodiesterase inhibitor sildenafil prior to a maximum exercise test at 5245 m lowered pulmonary artery systolic pressure and improved maximum exercise capacity compared to placebo, but other studies have not confirmed this. Alternatively sildenafil improves arterial oxygenation, which may explain the greater exercise capacity. Thus, it remains unresolved whether the rise in pulmonary artery pressures contributes to limiting exercise capacity at high altitude.
Exercise Performance at High Altitude
The effect of altitude on exercise performance varies based on the type of activity and the duration of stay at high altitude. Performance for running events of less than 2 minutes’ duration at less than 3000 m altitude is not impaired and may even be slightly improved because of decreased air density and resistance. Performances in events of longer duration are predictably diminished and can be restored only part way to sea-level capacity with prolonged stays at high altitude. Finally, as noted earlier, maximum oxygen consumption, peak heart rate, and peak minute ventilation are reduced at high altitude. Regardless of the duration of stay or the intensity of training, these values do not return to sea-level values.
Training at High Altitude
There is considerable interest in whether living and training at high altitude improves performance at lower elevations. In the past, many elite endurance athletes relocated to and trained at moderate altitudes of 2000 to 2500 m, but the beneficial effects on performance after return to sea level were generally not realized, because athletes could not attain the same maximum speeds and power output during training at altitude that they needed to reach during their sea-level races. More recently, interest has been raised in using intermittent hypoxic exposure as an alternative means to apply the benefits of high-altitude acclimatization to sea-level performance. For example, Beidleman and colleagues found that intermittent exposure to 4300-m altitude for 3 weeks improved speed and endurance in trained cyclists. In their landmark study, Levine and coworkers studied four well-matched groups of athletes: (1) those who lived low and trained low (1350 m), (2) those who lived low and trained high (3000 m), (3) those who lived high and trained high, and (4) those who lived high and trained low. The group that lived high and trained low seemed to have gained the benefit of hypoxic conditioning while not losing the intensity of training sessions. This advantage was presumably due to the modestly elevated hemoglobin from the altitude exposure, although there was individual variability in the erythropoietic response that correlated with improvement in performance.
Because of the limited access to high altitude for many athletes, recent interest has focused on “bringing the mountain to the athlete” by having the athlete spend time each day in oxygen-depleted tents that can be set up in their home or training center. The key question that has emerged from this practice and the studies noted, however, is this: What “dose” of hypoxic exposure and what level of athletic capacity is necessary to see a benefit in sea-level performance? A review of studies on the topic concluded that athletes needed to reside at 2000-2500 m for at least 4 weeks for at least 22 hours/day in order to derive physiologic benefit from the live high–train low model. However, a recent placebo-controlled trial in which elite athletes were blinded to whether they were in the “live high” group found no benefit to this strategy.
Maladaptation
The physiologic adaptations described earlier give most individuals the ability to tolerate high-altitude exposure. Regardless of the ultimate altitude achieved, however, individuals who ascend too rapidly to altitudes above 2500 m may display maladaptive responses and develop one of three forms of acute altitude illness: AMS, HAPE, and high-altitude cerebral edema (HACE). Because the risk of such illnesses decreases as individuals spend more time at altitude, long-term residents are not susceptible to these problems. However, several forms of chronic altitude illness, chronic mountain sickness and right heart failure, can affect these populations and represent important public health concerns.
Problems of Lowlanders on Ascent to High Altitude
Susceptibility to Altitude Illness
The incidence and severity of acute altitude illness depend on several factors, including the rate of ascent, the length of stay, and individual susceptibility. A trip to and from high altitude on the same day poses much less risk than an overnight stay because hypoxia is accentuated during sleep and because more prolonged exposure is associated with the overnight stay. Susceptibility to altitude illness varies considerably among individuals but, for a single individual, the symptoms are often reproducible given the same rate of ascent and other circumstances. Whereas men and women are equally susceptible to AMS, younger adults and the obese may be slightly more vulnerable. Recent trips to high altitude and residence at moderate altitude (>1900 m) have a protective effect. Of note, being in good physical condition does not exert any protective effect. There are insufficient data regarding whether preexisting chronic illnesses increase susceptibility.
At present, there are no adequate means to predict who will get sick upon ascent to high altitude. Variables such as the HVR and arterial oxygen saturation during hypoxic exposure have been proposed as useful predictors for developing AMS, but have not been validated in further studies, while specific genetic markers that reliably predict susceptibility have also yet to be identified. Richalet and colleagues have proposed a model to identify patients with risk factors for severe high-altitude illness, but this prediction tool requires the use of cardiopulmonary exercise testing under hypoxic conditions to identify susceptible individuals and, as a result, can be difficult to implement on a wide basis.
HAPE-susceptible individuals have been shown to have intense HPV and exaggerated pulmonary vascular responses to resting hypoxia and normoxic exercise, but this relationship has not been validated in prospective studies. In the end, the only useful predictive tool remains the patient’s prior experience at high altitude, particularly when the ascent rate and altitude of the prior and planned sojourns are similar.
Acute Mountain Sickness
AMS is the most common form of acute altitude illness, affecting 22% to 50% of travelers to altitudes between 1850 and 4240 m. Because of increasing awareness of the risks of altitude illness among the general population, incidence rates have likely fallen in commonly traveled regions, but very high incidence rates (up to 70%) continue to be reported with rapid ascents to high elevations in certain regions such as Mt. Kilimanjaro.
Clinical Presentation.
AMS is marked by the presence of headache plus one or more other symptoms including lassitude, insomnia, anorexia, nausea, dizziness, and, in severe cases, vomiting, although recent debate has developed as to whether headache is required for diagnostic purposes. Symptoms do not start immediately upon ascent and, instead, come on over many hours following arrival at altitudes above 2400 m (8000 feet), with the elevation at which symptoms begin varying significantly between individuals. Although many people complain of a sense of oppression in the chest, there is rarely any respiratory distress when the patient is at rest.
There are no characteristic physical examination findings in AMS, although crackles on auscultation and peripheral edema have been documented in some reports. No consistent changes are seen in body temperature, with some studies reporting a mild increase in temperature and others reporting a decrease of 1.7° C with no change in metabolic rate. Oxygen saturation values may be lower in individuals with AMS compared to those who remain healthy, but AMS can still be seen in those with what would be considered normal oxygenation at a particular altitude.
The symptoms and signs of AMS are nonspecific and other causes must be considered on the differential diagnosis, including dehydration, hypothermia, hyponatremia, exhaustion, alcohol hangover, carbon monoxide poisoning, and respiratory or cerebral infections. The diagnosis is based solely on the clinical history and there are no confirmatory laboratory tests. For a diagnosis of AMS and not the more severe HACE, the patient must have normal neurologic and mental status examinations.
Natural Course of Untreated AMS.
In a study of Indian soldiers airlifted to 3300 to 5500 m, incapacitating illness lasted 2 to 5 days but full recovery often took longer; 38% recovered fully within 3 days, 40% were still ill after 1 week, and 13% had symptoms after 1 month. In tourists sleeping at a lower altitude (2700 m), symptoms lasted an average of 15 hours (range: 6 to 94 hours). Most individuals tolerate or treat their own symptoms as the illness resolves over 1 to 3 days, but some persons with AMS seek medical help or are forced to descend. HACE or HAPE develops in a small percentage of those with AMS, especially when ascent is continued despite illness.
Pathophysiology.
Current concepts of pathophysiology emphasize the cerebral etiology of AMS. In severe illness, elevated intracranial pressure and cerebral edema have been noted on neurologic imaging and brain biopsy. Brain MRI studies have demonstrated swelling in the white matter and none in the gray matter in patients with HACE, a finding that suggests the edema is vasogenic in origin and initially confined to the white matter. Brain MRI also shows slightly increased brain volume and decreased intracerebral CSF volume in patients with AMS than in those without symptoms. Subsequent studies revealed conflicting data, with some reporting no evidence of changes consistent with cerebral edema and others showing evidence of mild increases in brain volumes and evidence of cytotoxic edema in subjects with mild to moderate AMS. It has been suggested that development of AMS may depend to some extent on the ability to shift CSF out of the cranium, and those individuals with smaller ventricles and CSF spaces may be at a disadvantage. This argument, referred to as the “tight-fit” hypothesis, might explain the increased susceptibility of younger persons, because age-related increases in the volume of sulci in older persons may provide more room to accommodate mild brain swelling.
Although evidence suggests that cerebral edema plays a role in severe AMS, it remains unclear whether this causes milder forms of the disease. There is only one study of intracranial pressure in subjects with early AMS, and it failed to demonstrate an increase in CSF pressure. Because headaches arise early in AMS at a time when edema and significant increases in intracranial pressure are unlikely, alternative explanations should be considered. The classic explanation that the headache and other symptoms of AMS are due to cerebral vasodilation alone seems unlikely, because the severity of AMS symptoms has not been shown to correlate with middle cerebral artery blood flow velocity. Limited evidence that sumatriptan, a 5-HT 1 receptor agonist, can prevent AMS or treat high-altitude headaches suggests that abnormal cerebrovascular reactivity or neuropeptide responses play a role in AMS pathophysiology. Another explanation is that hypoxia-induced changes in the blood-brain barrier alter permeability to plasma compounds with potential neurotoxicity or irritation. In studies in animals and cell cultures, hypoxia has been shown to mediate increased permeability of the blood-brain barrier to large molecules, but this has not been observed in humans. The mechanism underlying these blood-brain barrier changes is unclear, but factors such as oxygen free radical species and vascular endothelial growth factors have been proposed as possible contributors.
The findings in severe AMS of possible cerebral edema, peripheral edema, and proteinuria also suggest that there may be a defect in salt and water homeostasis and an increase in capillary permeability. AMS-susceptible individuals have elevated aldosterone and antidiuretic hormone levels when compared with controls, while persons acclimatizing well exhibit diuresis, net water loss, and low antidiuretic hormone values. A relatively recent study confirmed the findings regarding antidiuretic hormone but found no difference in aldosterone levels between healthy controls and those with AMS. These alterations in hormone levels cannot be attributed to a generalized increase in systemic capillary permeability or endothelial cell dysfunction, in that subjects with and without AMS demonstrate no differences in the global transvascular escape of labeled protein.
Hypoventilation may also contribute to the development of AMS. Acetazolamide and theophylline, two medications with respiratory stimulant properties, prevent the disorder and a brisk increase in ventilation on ascent to altitude has been associated with a lower incidence of AMS. At the same time, however, prospective studies have not demonstrated a correlation between a lower HVR measured before ascent and the development of AMS at high altitude. Bartsch and coworkers demonstrated that relative hypoventilation developed in subjects with early AMS compared to well-adapting subjects, suggesting that the blunted HVR was a response to AMS rather than a cause.
Prevention.
Appropriate pharmacologic and nonpharmacologic measures to prevent or ameliorate AMS have been described in recently published guidelines. Because overly rapid ascent to high elevation is the leading risk factor for altitude illness, gradual ascent, with rest days for acclimatization, is considered the single best way to prevent all forms of altitude illness. It is recommended that above 3000 m, individuals should increase their sleeping elevation by no more than 300 to 500 m per night and should take an acclimatization day and sleep at the same elevation a second night every 3 to 4 days or after any large gain in elevation. Only a single study has attempted to study the benefits of slow ascent in a randomized, controlled manner, and strict adherence to published recommendations may be difficult due to a variety of logistical factors. Travelers should avoid overexertion. They should refrain from heavy alcohol consumption or narcotic medication use, particularly before bedtime, in order to avoid hypoventilation, which can exacerbate the hypoxemia of high altitude. These strategies have never been subjected to rigorous study but represent common sense measures in light of the known physiologic changes at altitude.
Pharmacologic means can also be used to prevent AMS. The most commonly used medication for this purpose is acetazolamide. Its principal action is renal carbonic anhydrase inhibition, which causes a bicarbonate diuresis and a metabolic acidosis, which, in turn, stimulates ventilation, increases alveolar P o 2 , and enhances oxygen off-loading in the tissues. In effect, the drug speeds natural acclimatization by accelerating the pace of ventilatory adaptation by several days. Actions of acetazolamide on carbonic anhydrase elsewhere in the body, such as the peripheral and central chemoreceptors, may also contribute to its preventive role.
Debate persists about the proper dose of acetazolamide, but most published resources continue to recommend a dose of 125 to 250 mg twice a day ( Table 77-2 ). Acetazolamide is well tolerated with the most common side effect being paresthesias involving the hands, feet, and lips. There may be nausea and drowsiness, and carbonated beverages may taste flat, probably secondary to the inhibition of hydration of carbon dioxide on the palate. Acetazolamide has a sulfonamide moiety and shares the cautions common to all sulfa drugs. The likelihood of a cross reaction is low (approximately 7% to 10%), but case reports describe fatal anaphylactic reactions in people with documented sulfa allergy who received acetazolamide.
Medication | Dose in Normal Individuals | Liver Disease | Chronic Kidney Disease | Other Major Dosing Issues |
---|---|---|---|---|
Acetazolamide | AMS prevention: 125 or 250 mg bid AMS treatment: 250 mg bid | Use is contraindicated | Avoid use in patients with GFR < 10 mL/min, metabolic acidosis, hypokalemia, hypercalcemia, and hyperphosphatemia or recurrent nephrolithiasis | Avoid in patients on long-term high-dose aspirin or with ventilatory limitation (FEV 1 < 25% predicted) Caution in patients with documented sulfa allergy Avoid concurrent use of topiramate |
Dexamethasone | AMS prevention: 4 mg bid or 2 mg qid HACE treatment 8 mg once followed by 4 mg every 6 hours | No contraindication; no dose adjustments necessary | No contraindication; no dose adjustments necessary | Expect elevated blood glucose values when used in diabetic patients Avoid in patients at risk for peptic ulcer disease or upper gastrointestinal bleeding Caution in patients at risk for amebiasis or strongyloidiasis |
Nifedipine | HAPE prevention: 20 to 30 mg of sustained release version every 12 hours HAPE treatment: 20 to 30 mg of sustained release version every 12 hours | Reduced dose required (10 mg bid) | No contraindication; no dose adjustments necessary | Caution in patients taking medications metabolized by CYP450 3A4 and 1A2 pathways Caution during concurrent use with other antihypertensive medications |
Tadalafil | HAPE prevention: 10 mg bid HAPE treatment: Unknown | Child’s class A and B: Maximum 10 mg daily Child’s class C: Do not use tadalafil | Dose adjustments necessary if GFR < 50 mL/min. If GFR 30-50 mL/min, use 5-mg dose, maximum 10 mg in 48 hr. If GFR < 30 mL/min, no more than 5 mg | Increased risk for gastroesophageal reflux Caution in patients taking medications metabolized by CYP450 3A4 pathway Avoid concurrent use of nitrates or α-blockers |
Sildenafil | HAPE prevention: 50 mg every 8 hours HAPE treatment: Unknown | Dose reductions recommended. Starting dose 25 mg tid Avoid use in patients at risk for variceal bleeding | Dose adjustments necessary if GFR < 30 mL/min | Increased risk for gastroesophageal reflux Caution in patients taking medications metabolized by CYP450 3A4 pathway Avoid concurrent use of nitrates or α-blockers |
Salmeterol | HAPE prevention: 125 µg bid HAPE treatment: No established role in HAPE treatment | Insufficient data | No contraindication; no dose adjustments necessary | Avoid concurrent use of β-blockers, monoamine oxidase inhibitors or tricyclic antidepressants Possible adverse effects in coronary artery disease patients prone to arrhythmia |
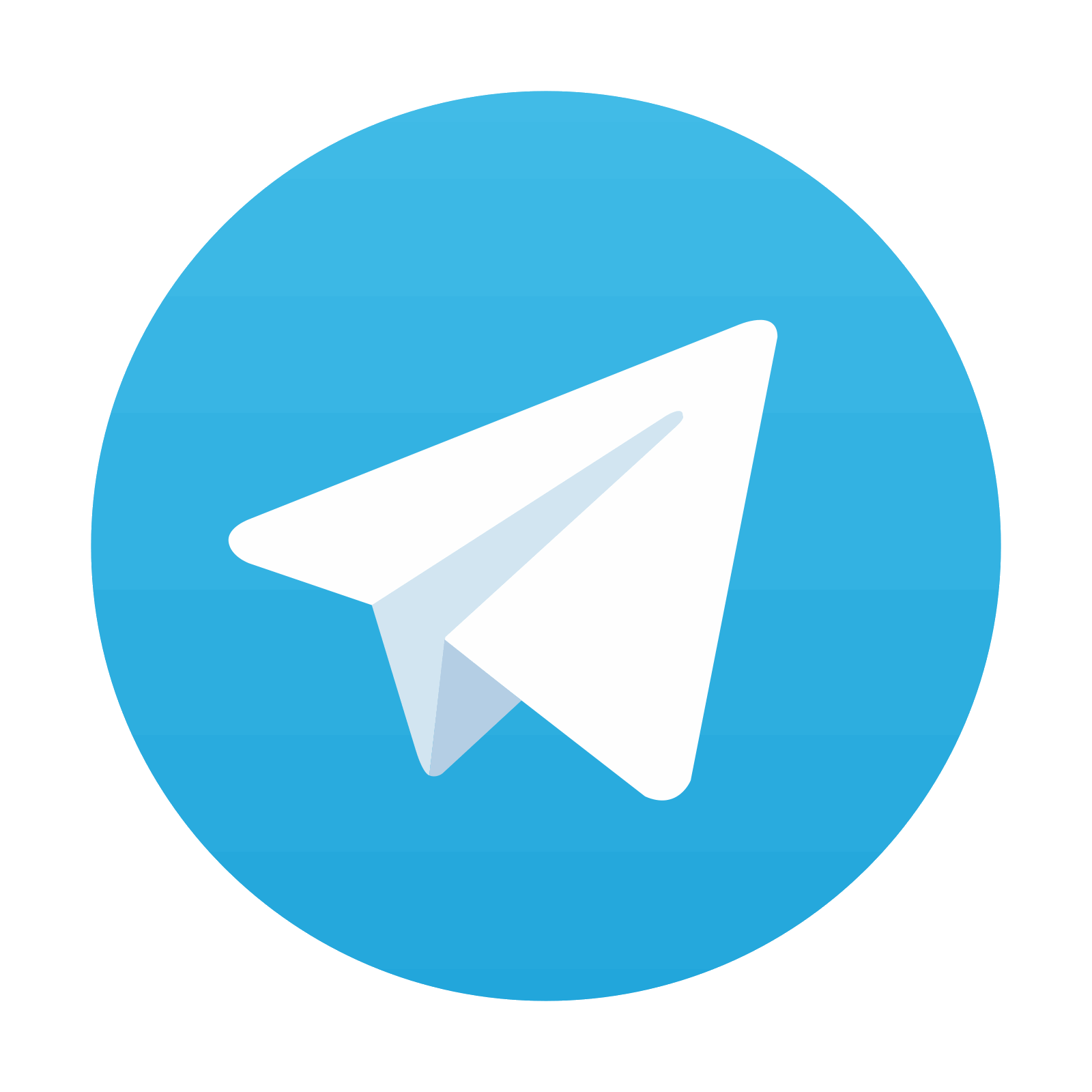
Stay updated, free articles. Join our Telegram channel
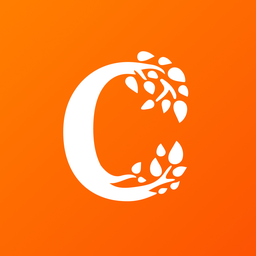
Full access? Get Clinical Tree
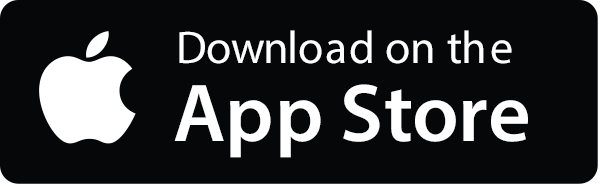
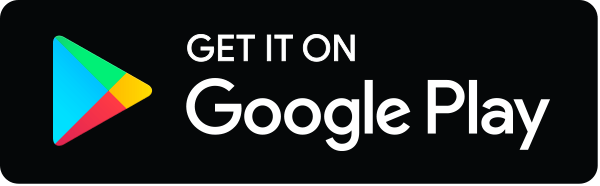
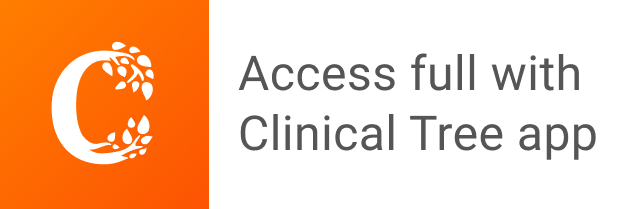