Fig. 21.1
Adenosine triphosphate (ATP) . ATP is the major source of chemical energy used to power the reactions that support contractile and other processes in myocardium and all other living tissues. The ~ symbol is used to designate a phosphate bond which has a very high level of stored chemical energy. Although ATP contains two ~ phosphate bonds, it is the hydrolysis of the terminal phosphate bond that releases the energy that directly powers cellular processes

In the myocardium, as in other biologic tissues, most energy-driven processes use ATP as the immediate source of energy. Hydrolysis of the terminal phosphate bond of ATP releases energy that can be captured and used to drive (energy-dependent) processes such as protein synthesis, muscle contraction, ion transport, etc. Therefore, the ability of the heart to pump blood to the pulmonary and systemic circulations is dependent on the presence of adequate concentrations of ATP. Unfortunately, myocardial stores of ATP are modest in relation to the rate of expenditure, and continuous ATP synthesis is required to support energy-requiring processes. Importantly, most of the energy required for ATP synthesis is derived from the controlled breakdown of the chemical bonds in carbohydrates (glucose) and fatty acids; body protein-derived amino acids are used to fuel ATP generation only when supplies of the major fuels are compromised, as would be the case during a period of starvation.
To summarize, this chapter will describe: (1) how carbon substrates and oxygen are delivered to the heart, (2) the biochemical pathways within the heart (most of the same pathways are present in all tissue types) that transfer the chemical bond energy stored in carbon substrates to ATP, (3) some of the ways that these pathways are regulated such that they are capable of responding to the increased ATP demand associated with increased cardiac work states, and (4) some of the alterations in these processes that occur under changing physiological states in normal and diseased hearts. The reader should understand that this relatively brief summary of myocardial metabolism is, by necessity, an extremely superficial overview of the individual topics. For those readers wishing to review some of the major topics in greater detail, a list of recent references—primarily current topical reviews, research reports, and a standard biochemistry textbook—is provided at the end of the chapter.
21.2 Myocardial Blood Flow: Carbon Substrate and Oxygen Delivery to the Heart
Blood containing carbon substrates and oxygen is delivered into human and animal hearts (see Chap. 6) by coronary arteries that originate from the proximal aorta. These arteries then subdivide to form progressively smaller branches that arborize inward throughout the ventricular wall and supply blood to the myocardium (Fig. 21.2). The left ventricular wall (for descriptive purposes) is arbitrarily subdivided into transmural layers termed the subepicardium (outermost layer), the midmyocardium, and the subendocardium (innermost layer). The coronary arterial tree terminates in muscular vessels 60–150 mm in diameter termed arterioles. The arterioles are the major locus of resistance to blood flow, and contraction or relaxation of the smooth muscle in the walls of the arterioles (vasomotion) provides the mechanism for control of the rate of blood flow into the myocardium. Each arteriole supplies an array of capillaries, thin-walled tubes comprised of a single layer of endothelial cells, across which most of the exchange of nutrients, oxygen, and metabolic waste products occurs. As their terminal end capillaries coalesce into venules, the initial component of the cardiac venous system then conducts most postcapillary blood back into the cardiac chambers, primarily through the coronary sinus that drains into the right atrium. For more details on the coronary circulation, see Chap. 8.
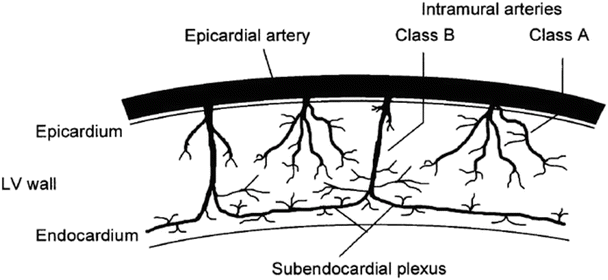
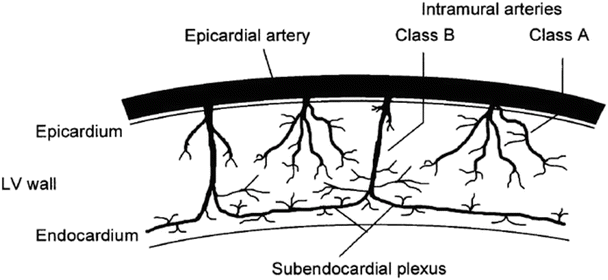
Fig. 21.2
Transmural distribution of the coronary arterial system . The large epicardial conductance arteries supply shallow and deep branches to the subepicardium and subepicardium, respectively. These perforating vessels arborize to create the arteriolar network that supplies the myocardial capillary bed. LV left ventricle. Reprinted from Duncker DJ and Bache RJ, Regulation of coronary vasomotor tone under normal conditions and during acute myocardial hypoperfusion, Pharmacol Ther, Vol. 86, 87–110, 2000. Pharmacology and Therapeutics (by International Union of Pharmacology). Reproduced with permission of Elsevier Inc., in the format Book via Copyright Clearance Center
21.2.1 Regulation of Myocardial Blood Flow
Coronary blood flow is highly regulated and falls or rises appropriately in response to subtle or large changes in the rate of myocardial energy expenditure. The principal purpose of the heart is to generate the pressure that forces blood from the ventricles into the pulmonary and systemic circulations. Elevation of the cardiac rate of ATP expenditure during exercise or other stresses increases myocardial demand for oxygen and carbon substrates. In clinical practice, there is often a need to assess the effects of changes of the rate of myocardial energy expenditure on cardiac performance (e.g., during exercise stress testing). Since routine direct measurement of myocardial oxygen consumption is not practical, a rough estimate of the change in the energy demands of the heart is afforded by the product of systolic blood pressure multiplied by the times per minute that pressure generation occurs (heart rate); this is termed the rate–pressure product . This measurement provides a simple estimate of changes in the metabolic requirement of the heart resulting from different cardiac work states in animals and humans.
The coronary circulation operates on the principle of “just-in-time” delivery of oxygen and carbon substrates. In other words, coronary blood flow is regulated to be only minimally greater than required to meet the instantaneous metabolic demands of the heart. Furthermore, the heart extracts 70–80 % of the O2 from the blood as it flows through the coronary capillaries. Because of this high level of basal oxygen extraction, there is little ability to increase oxygen uptake by means of increased extraction of oxygen from the blood. As a result, increases in myocardial energy requirement during exercise or other stresses must be satisfied by concomitant increases of coronary blood flow. As noted, ATP and oxygen stores in the myocardium are relatively small. Therefore, the response time for the increase in coronary flow following an increase in cardiac work must be rapid (i.e., a few seconds). From these considerations, it is clear that highly responsive signaling systems exist which link the rate of myocardial energy expenditure to vasomotor activity in the resistance vessels that control coronary blood flow. Interestingly, these signaling systems are not fully understood despite intense study over the last 100 years.
21.2.2 Signaling Pathways Regulating the Coronary Circulation
The regulatory signals that increase blood flow in response to increases/decreases of cardiac work can be classified as having feedback or feed-forward characteristics; the final common response to these signals is relaxation/contraction of vascular smooth muscle cells within the arteriolar resistance vessels that control coronary blood flow (Fig. 21.3). Several major feedback mechanisms resulting from increased cardiomyocyte metabolism (including adenosine, nitric oxide, ATP, H2O2, and others) cause opening of ATP-sensitive potassium channels (KATP) located within the sarcolemma of arteriolar smooth muscle cells. Opening of these channels allows potassium to escape from the cytosol of the smooth muscle cells, resulting in hyperpolarization (increased negativity) of the arteriolar smooth muscle cell membrane. The increased negativity of the membrane causes sarcolemmal voltage-dependent calcium channels to close; as a result, calcium entry into the smooth muscle is reduced, the vessel relaxes (i.e., dilates), and coronary blood flow increases. In addition to effects on the KATP channels, adenosine (a product of ATP utilization in the cardiomyocyte) also has potent, direct dilator effects on arteriolar smooth muscle. Another metabolic feedback signal is nitric oxide generated by the vascular endothelium. Mechano-transduction of flow-induced shear forces exerted on the endothelial cells augments nitric oxide synthesis. In addition to causing potassium channel opening, nitric oxide also initiates direct relaxation processes in vascular smooth muscle. It is important to note that this brief discussion does not include many of the known feedback mechanisms involved in regulation of coronary blood flow.
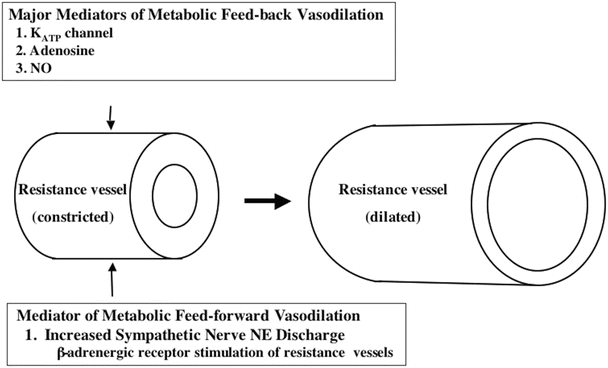
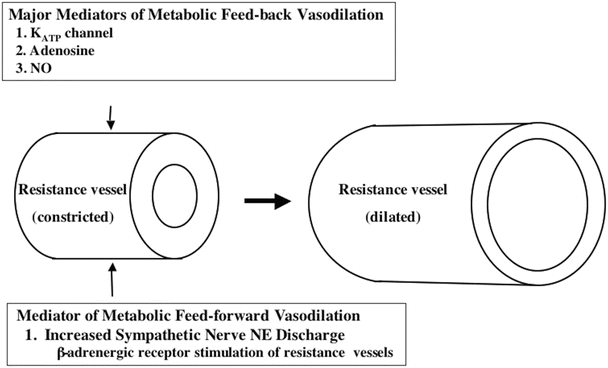
Fig. 21.3
Major feedback and feed-forward mechanisms underlying metabolic vasodilatation of resistance vessels are depicted (see text for discussion). K ATP channel ATP-inhibited potassium channel, NO nitric oxide
Notably, increased cardiac sympathetic nerve activity (i.e., during exercise) activates a feed-forward mechanism for control of coronary blood flow which augments the local metabolic vasodilator influences. The sympathetic neurotransmitter norepinephrine activates α- and β-adrenergic receptors located within the sarcolemma of arteriolar smooth muscle cells. Activation of similarly located α-adrenergic receptors causes modest constriction of the large coronary arteries; since these arteries function as conduit vessels that offer little resistance to blood flow, this has little effect on coronary flow. However, activation of arteriolar β-adrenergic receptors results in relaxation (vasodilation) of these small resistance vessels; the resultant decrease of coronary resistance causes a feed-forward increase in blood flow that is independent of local metabolic mechanisms and augments the increase of coronary blood flow during exercise.
Pharmacologic studies have shown that simultaneous blockade of the coronary KATP channels, adenosine, and nitric oxide pathways significantly decreases myocardial blood flow in the resting animal. Moreover, the increase of coronary flow that normally occurs during exercise is severely blunted, resulting in a perfusion-metabolism mismatch that is accompanied by evidence of ischemia even in the normal heart. Hence, activation of these three pathways appears to be the primary means by which metabolic vasodilatation is achieved in the heart. However, in the healthy heart, blockade of any one of these mechanisms for smooth muscle relaxation will elicit compensatory (i.e., increased) activation of the other pathways to minimize changes in coronary blood flow. Lastly, other (not discussed) circulatory regulatory mechanisms may also be of biological significance.
21.2.3 Blood Flow in the Diseased Heart
Coronary blood flow in the diseased heart can be limited by: (1) partial or complete obstruction of the large coronary arteries (e.g., atherosclerotic disease), (2) decreased responsiveness of the signaling systems relating blood flow to energy requirements, and/or (3) increases in extravascular mechanical forces acting to compress the small vessels in the wall of the left ventricle. In the case of obstructive (generally atherosclerotic) disease of the epicardial coronary arteries, a moderately narrowed vessel may only restrict blood flow during periods of increased blood flow demand (i.e., it reduces vasodilator reserve), while a severely narrowed vessel may limit blood flow even when the subject is at rest. In the presence of a moderate coronary obstruction, the arteriolar bed can maintain adequate blood flow by metabolic signaling-based arteriolar vasodilatation. That is, a decrease in small vessel resistance can compensate for the increased resistance caused by a proximal coronary artery stenosis. However, when the capacity for vasodilation of the arterioles has been exhausted, any further increase in cardiac work cannot induce an increase of blood flow, and the myocardium supplied by the narrowed epicardial vessel will become ischemic. There is also considerable evidence that malfunction of metabolic signaling pathways in the arteriolar resistance vessels (e.g., in the absence of obstructed large coronary arteries) can cause myocardial ischemia in certain patients.
In the normal heart, blood flow to the inner layers of the left ventricle occurs principally during diastole. This is because tissue pressures in the wall of the left ventricle during systole are so great that inner layer arterioles are squeezed shut by the extravascular compressive forces produced by cardiac contraction. Diastolic left ventricular tissue pressures are also greatest in the subendocardium. When the heart fails and/or becomes hypertrophied, these extravascular compressive forces increase as left ventricular filling pressure increases. In the hypertrophied or failing heart, slowing of myocyte relaxation also shortens the duration of the diastolic interval and thus limits coronary flow reserve in the inner cardiac layers. In the normal heart, autoregulatory (i.e., metabolic vasodilatation) processes cause enough arteriolar vasodilation in the subendocardium to compensate for systolic underperfusion. However, increased left ventricular diastolic pressure (and myocardial tissue pressure) in the failing heart may compress the arteriolar bed in the inner myocardial layers sufficiently to overwhelm autoregulatory mechanisms, particularly those that normally maintain adequate subendocardial blood flow. Since subendocardial blood flow occurs almost exclusively during diastole, tachycardia also acts to impede blood flow in the subendocardium by shortening of the diastolic interval. Thus, even in the absence of obstructive coronary artery disease or intrinsic arteriolar abnormalities, increased arteriolar compression can limit blood flow to the inner myocardial layers of the diseased heart. Importantly, if these abnormalities limit substrate delivery to the inner myocardial layers, they will disrupt the balance between ATP synthetic capacity and ATP utilization in this region of the ventricular wall. Thus, the extravascular forces cause the subendocardium to be the region of the ventricular wall that is most vulnerable to hypoperfusion and ischemia.
21.3 Intermediary Metabolism and Bioenergetics in the Normal Heart
Glucose and fatty acids are the main substrates consumed by the heart, with fatty acid consumption predominating under most circumstances (Fig. 21.4). Exceptions to this statement will be discussed later.
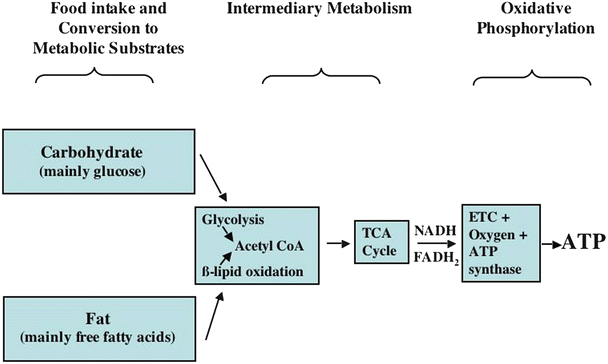
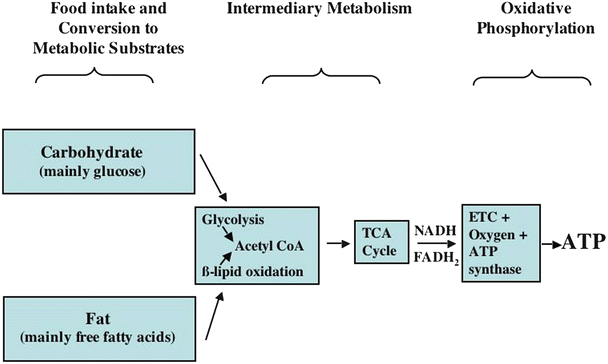
Fig. 21.4
A general overview of carbon substrate metabolism in the heart . First, ingested food is broken down into usable carbon substrates, primarily amino acids (not major contributors to ATP synthesis), glucose, and fatty acids. The pathways which convert amino acids and other molecules to glucose are not shown. Glucose and fatty acids are processed (via intermediary metabolic processes) to yield the reducing equivalents, NADH and FADH2, which supply the energy necessary to power oxidative phosphorylation. The latter process, which occurs within the mitochondria in the presence of oxygen, supplies almost all of the ATP synthesized and utilized in the heart. ATP adenosine triphosphate, ETC electron transport chain, FADH 2 flavin adenine dinucleotide, NADH nicotinamide adenine dinucleotide, TCA tricarboxylic acid
21.3.1 Glucose Metabolism
Figure 21.5 shows a flowchart for glucose metabolism. Glucose enters the cardiomyocyte via the sarcolemmal glucose transport proteins, GLUT 1 (that is insulin independent) and GLUT 4 (that is insulin dependent). Once in the cell, glucose is phosphorylated to glucose-6-phosphate by the enzyme hexokinase. Since glucose-6-phosphate is membrane impermeable, this effectively traps glucose within the cell. Glucose-6-phosphate can enter the glycogen synthesis pathway (glycogen is a macromolecular polymeric storage form of glucose), the pentose monophosphate shunt (that generates NADPH and ribose, moieties utilized in many important cellular processes), or it can undergo molecular rearrangement via the enzyme phosphohexose isomerase to form fructose-6-phosphate and continue on through the glycolytic series of reactions. A second phosphorylation of fructose-6-phosphate via phosphofructokinase generates fructose-1,6-bisphosphate. Each of these phosphorylations consumes one molecule of ATP. Fructose-1-6-bisphosphate is next split into glyceraldehyde-3-phosphate and dihydroxyacetone phosphate by the enzyme aldolase. These two molecules are in constant exchange with each other via the enzyme phosphotriose isomerase. Glyceraldehyde-3-phosphate is then phosphorylated to form 1,3-bisdiphosphoglycerate by the enzyme glyceraldehyde-3-phosphate dehydrogenase. The phosphate bond in the 1 position is a high-energy-containing bond (signified by the ~P symbol); this reaction also simultaneously reduces NAD+ to NADH. Cytosolic oxidation of NADH and transport of the two removed electrons and H+ into the mitochondrial matrix then occur in cardiac muscle via the malate-aspartate shuttle (not shown in Fig. 21.5). In the mitochondrial matrix, NAD+ is then reduced back to NADH which can be utilized by the mitochondria to generate ATP (to be discussed later). In the next cytosolic reaction, the high-energy phosphate bond in 1,3-bisdiphosphoglycerate is transferred to adenosine diphosphate (ADP) to form ATP and 3-phosphoglycerate via the enzyme phosphoglycerate kinase. The latter molecule is converted to 2-phosphoglycerate by the enzyme phosphoglycerate mutase. Enolase, another cytosolic enzyme, then converts 2-phosphoglycerate to the ~P-containing molecule phosphoenolpyruvate. The latter is converted to pyruvate by the enzyme pyruvate kinase. During this reaction, the ~P in phosphoenolpyruvate is transferred to ADP to form a second ATP molecule. Pyruvate can then enter the mitochondria to be further metabolized by the pyruvate dehydrogenase (PDH) complex of enzymes. Both the pyruvate dehydrogenase complex that converts pyruvate to acetyl-CoA (and NAD+ to NADH), and the tricarboxylic acid (TCA) cycle that metabolizes acetyl-CoA are located within the mitochondria (Figs. 21.5, 21.6a, and 21.7). Within the mitochondria another metabolic pathway for pyruvate metabolism also exists. The enzyme, pyruvate carboxylase, converts pyruvate to oxaloacetate, which is a TCA cycle intermediate (Figs. 21.6a and 21.8). The significance of the latter reaction will be discussed later.
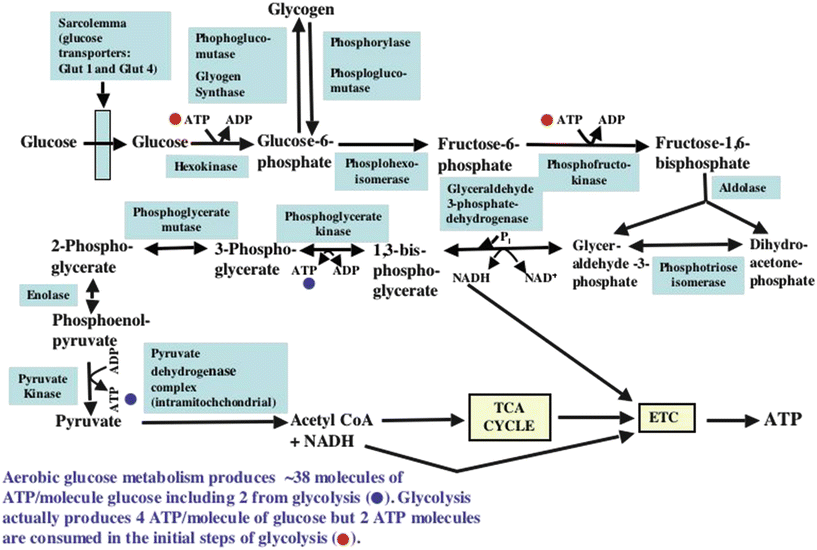
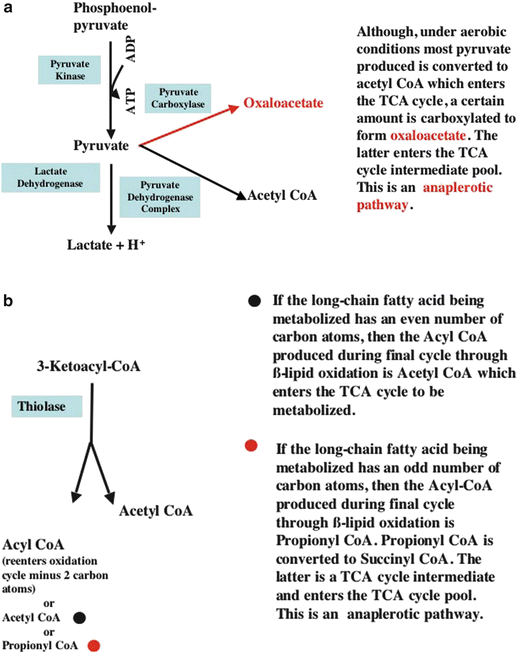
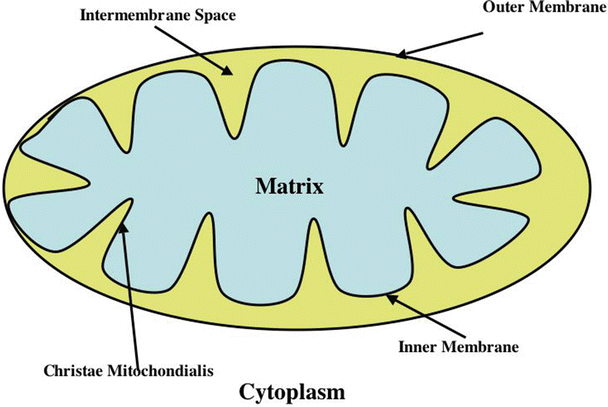
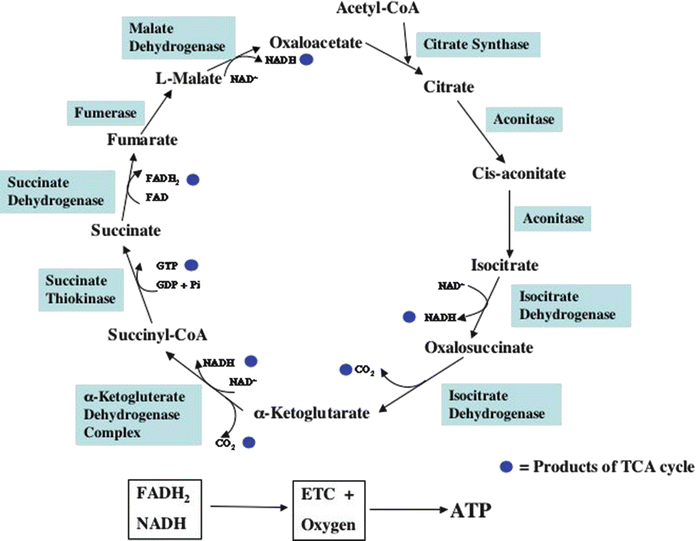
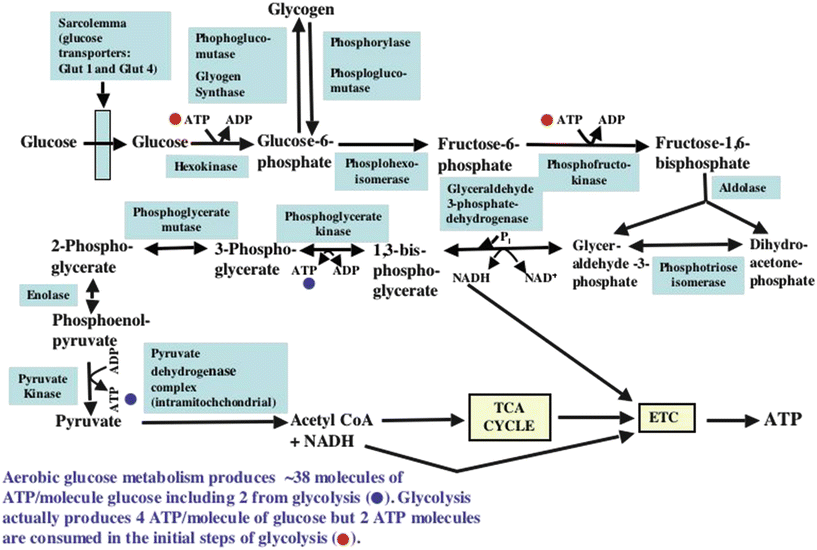
Fig. 21.5
Flowchart of cellular uptake of glucose and the pathways through which glucose metabolism proceeds. See text for discussion. ADP adenosine diphosphate, ATP adenosine triphosphate, ETC electron transport chain, NADH nicotinamide adenine dinucleotide, TCA tricarboxylic acid
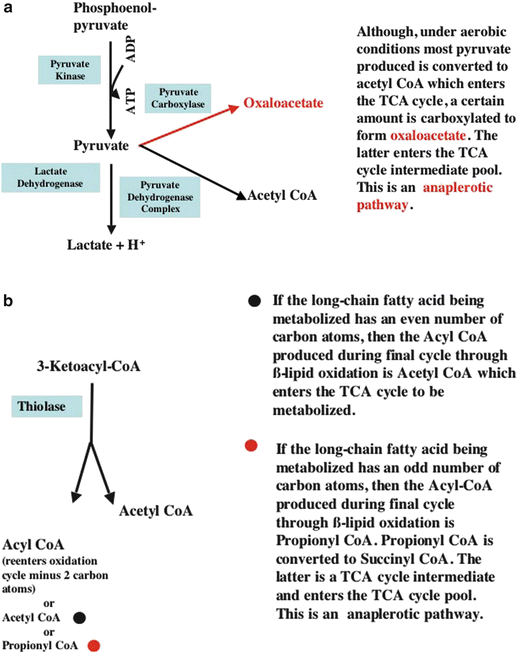
Fig. 21.6
Anaplerotic and cataplerotic processes. Anaplerotic process es are those which supply substrate used to maintain the TCA cycle intermediate pool size. In contrast, cataplerotic processes remove substrates from the TCA cycle intermediate pool and decrease its size. The balance between these two processes determines the TCA cycle pool size. (a) Shows how glucose (via its metabolic product, pyruvate) contributes to anaplerosis. (b) Shows how the β-oxidation of odd-numbered carbon chain fatty acids contributes to anaplerosis. (Note that readers may want to review Fig. 21.13, a flowchart for β-lipid oxidation, before examining (b) which depicts the terminal reaction of β-lipid oxidation.) ADP adenosine diphosphate, ATP adenosine triphosphate, TCA tricarboxylic acid
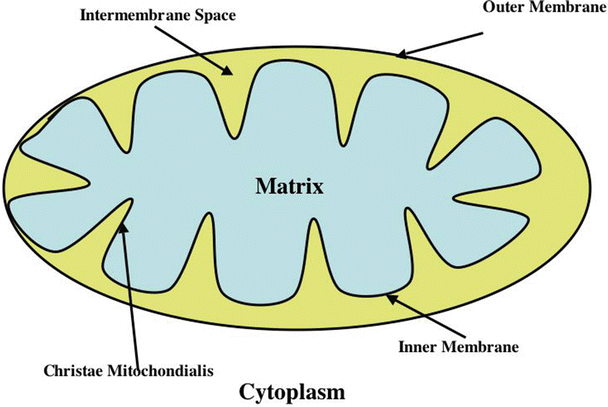
Fig. 21.7
Major features of mitochondrial morphology . See text for discussion
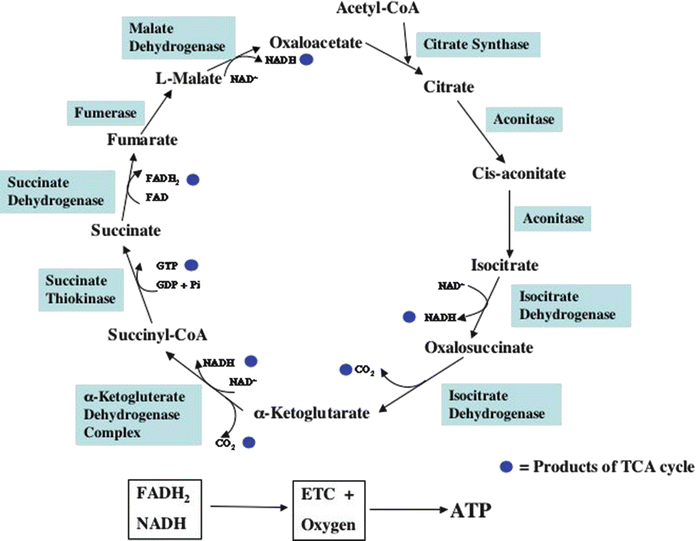
Fig. 21.8
Tricarboxylic acid (TCA) cycle . The filled blue circles are the products resulting from one turn of the TCA cycle. See text for discussion. ATP adenosine triphosphate, ETC electron transport chain, FADH 2 flavin adenine dinucleotide, NADH nicotinamide adenine dinucleotide, TCA tricarboxylic acid
Within the cytosol , pyruvate can be converted to lactic acid by lactic acid dehydrogenase (LDH). Lactate and a hydrogen ion are then exported from the cell via the monocarboxylic acid transporter (Figs. 21.5, 21.6a, and 21.9). This pyruvate to lactic acid reaction is associated with the oxidation of NADH to NAD+ and, as will be shown, is critical to maintaining glycolysis when the availability of oxygen to the cardiomyocyte is limited. Conversely, under aerobic conditions, lactate in the blood can be transported into the cardiomyocyte by the monocarboxylic acid transporter, to be converted to pyruvate by LDH. The LDH-catalyzed reaction also reduces NAD+ to NADH, and the reducing equivalents from NADH can be transferred into the mitochondrial matrix by the malate-aspartate shuttle, and the pyruvate generated is available for processing by PDH.
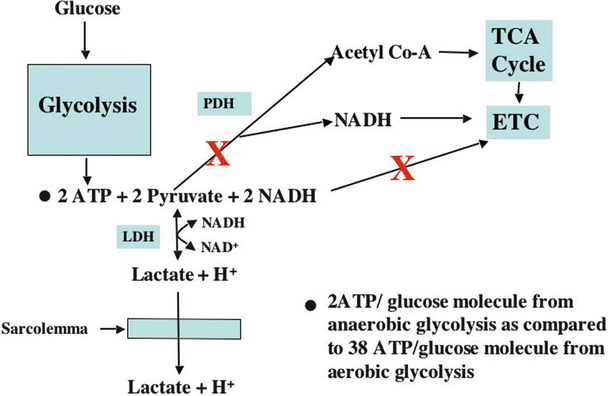
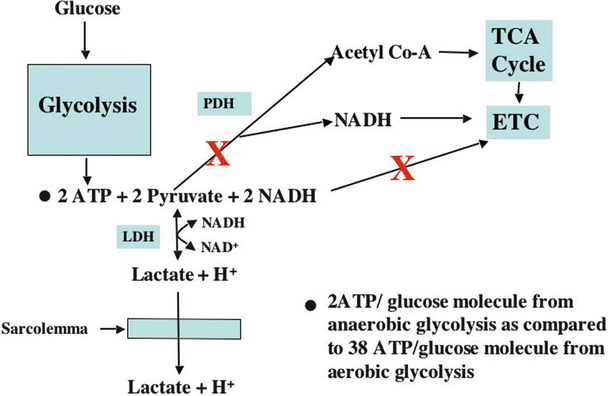
Fig. 21.9
Flowchart for anaerobic glucose metabolism . A large red X indicates metabolic pathways blocked during ischemia. See text for discussion. ATP adenosine triphosphate, ETC electron transport chain, LDH lactic acid dehydrogenase, NADH nicotinamide adenine dinucleotide, PDH pyruvate dehydrogenase, TCA tricarboxylic acid
During glycolysis of one glucose molecule, two pyruvate molecules, four ATP molecules, and two NADH molecules are produced. However, because two ATP molecules are consumed early in the glycolytic pathway, the net production of ATP in the cytosol is two molecules/glucose molecule. As previously indicated, pyruvate and NADH are utilized in the mitochondria for oxidative generation of ATP. Complete metabolism of one glucose molecule (i.e., including oxidation of the products of glycolysis in mitochondria) results in the formation of many additional ATP molecules (30–36, depending on the literature cited) than does glycolysis alone. However, under conditions when mitochondrial function is severely oxygen limited, the oxidative contribution to ATP synthesis is lost, and only two ATP molecules can be formed from each glucose molecule.
21.3.2 The Mitochondrion
Mitochondria, which are the primary site of ATP synthesis in most mammalian cells, contain the β-lipid oxidation pathway enzymes, the TCA cycle enzymes, the electron transport chain, and the F1F0-H+-ATPase (also called F1F0-ATP synthase or ATP synthase). To better understand the location of these systems, mitochondrial structure will be briefly reviewed (Fig. 21.7). The inner mitochondrial membrane contains the electron transport chain, F1F0-H+-ATPase, the adenine nucleotide translocase, and other transporters. The mitochondrial matrix contains the TCA cycle enzymes, β-lipid oxidation enzymes, and many other enzymes and reactants. The cristae are invaginations that markedly expand the surface area of the inner membrane and, thereby, the quantity of energy generation-associated proteins that can be contained within the inner membrane. The inner membrane-bound components of the metabolic pathways are positioned to optimize the flow of substrates through their reaction sequences. The mitochondrial outer membrane forms a boundary between the cellular cytoplasm and the mitochondrial intermembrane space. The intermembrane space contains creatine kinase which is important for high-energy phosphate transport out of mitochondria (Figs. 21.7, 21.10, and 21.11) and cytochrome c, a component of the electron transport chain. The importance of the intermembrane space to oxidative ATP synthesis will be discussed subsequently. The outer mitochondrial membrane also contains voltage dependent anion channels (VDAC) through which ATP and other moieties exit the intermembrane space and through which ADP and many other molecules enter the intermembrane space.
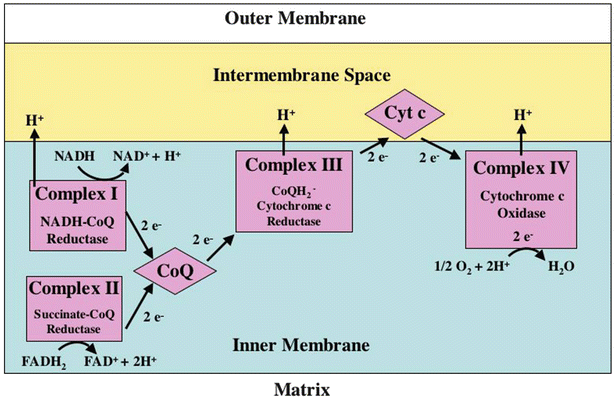
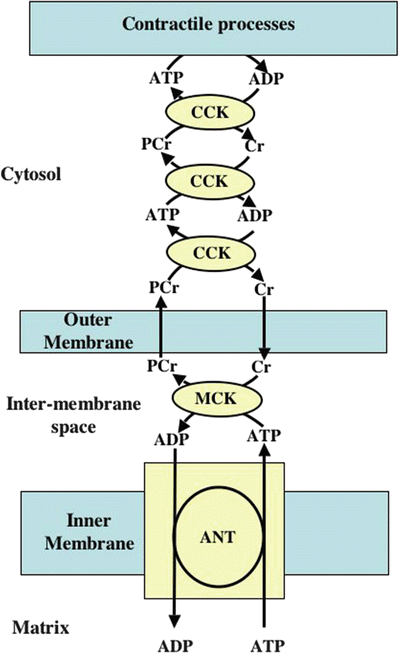
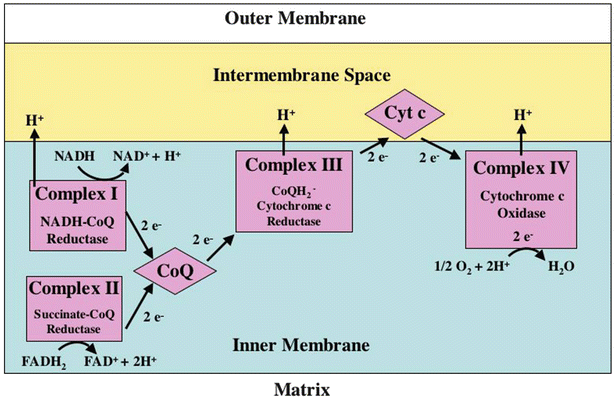
Fig. 21.10
Electron transport chain (ETC) . See text for discussion. FADH 2 flavin adenine dinucleotide, NADH nicotinamide adenine dinucleotide
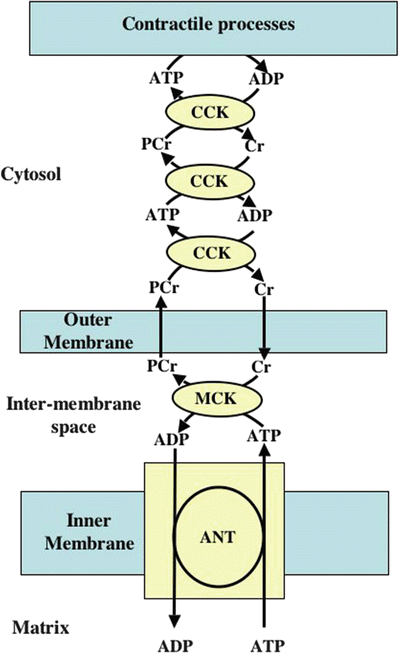
Fig. 21.11
Creatine kinase shuttle hypothesis . The diffusion rates of ATP out of the intermembrane space and through the cytosol and ADP through the cytosol and into the intermembrane space are considered to be relatively slow compared to those of creatine and creatine phosphate. The creatine kinase shuttle is thought to facilitate the transfer of ADP from sites of ATP utilizing reactions to the mitochondrial intermembrane space and also to facilitate the transfer of ATP to the sites of utilization. The way the shuttle is proposed to function is described below. The adenine nucleotide transporter (ANT) , which is not rate limiting, transports ADP from the mitochondrial intermembrane space to the mitochondrial matrix where it is rephosphorylated back to ATP. The ANT simultaneously transports newly synthesized ATP from the matrix to the intermembrane space. Within the intermembrane space, the mitochondrial isozyme of creatine kinase (MCK) transfers the terminal high-energy phosphate of ATP to creatine to form phosphocreatine. Phosphocreatine then diffuses into the cytosol, and the ADP produced by this reaction in the intermembrane space is returned to the mitochondrial matrix by ANT, where it is rephosphorylated. The phosphocreatine which has diffused into the cytosol is then used as a high-energy phosphate donor for the rephosphorylation of cytosolic ADP by the cytosolic isozyme of creatine kinase. Because the isozymes of creatine kinase have extremely rapid turnover rates, they can facilitate transport of ATP (by, in effect, shuttling the terminal high energy phosphate bonds) from the mitochondria to the sites of ATP hydrolysis and of ADP from ATP hydrolysis sites back to the mitochondrial outer membrane. There, phosphocreatine leaving the mitochondria is used to rephosphorylate ADP, and the creatine liberated from phosphocreatine diffuses into the mitochondrial intermembrane space to be rephosphorylated. Obviously, ATP and ADP also pass through the VDAC, but at slower rates than creatine and phosphocreatine. As a result, cytosolic ADP levels (including those in proximity to the points of ATP utilization) are kept at low levels even if the rate of ATP utilization increases. The stability of ADP levels in the face of increasing ATP utilization permits ATP to maintain a high level of free energy that can be transferred to energy-requiring cellular processes. ADP adenosine diphosphate, ANT adenine nucleotide transporter, ATP adenosine triphosphate, CCK cytosolic creatine kinase, Cr creatine, MCK mitochondrial creatine kinase, PCr phosphocreatine
21.3.3 Fatty Acid Metabolism
Figure 21.12 presents a flowchart for the β-lipid oxidation pathway. Dietary long-chain, nonesterified, free fatty acids (NEFAs) are generally the predominant carbon substrate in normal myocardium. They are transported in the blood bound to plasma albumin, lipoprotein moieties, or in the form of triacylglycerol which is also bound to albumin. The latter can be broken down to release NEFAs by an enzyme present in the plasma and at the surface of the capillary and cardiomyocyte. After dissociating from albumin, NEFAs are transported accross capillary walls and into cardiomyocytes by fatty acid transport proteins. Within the cell, NEFAs are bound to fatty acid-binding proteins which provide solubility and intracellular transport. Once in the cell, NEFAs are either reesterified and stored as triglycerides or activated by acyl-CoA synthetase (which requires the presence of free CoA and ATP) to form a long-chain acyl-CoA. Because long-chain acyl-CoA cannot readily diffuse through the mitochondrial inner membrane, it is converted to long-chain acyl carnitine by carnitine palmitoyltransferase 1 at the outer surface of the inner mitochondrial membrane and then transported across the inner membrane by carnitine-acylcarnitine translocase (which also transports free carnitine liberated by carnitine palmitoyltransferase 2 back into the intermembrane space; see below). The long-chain acyl carnitine is next converted back to acyl-CoA at the inner surface of the mitochondrial inner membrane by carnitine palmitoyltransferase 2. Long-chain acyl-CoA is then processed by a sequence of enzyme-catalyzed reactions that comprise the β-lipid oxidation pathway. If the long-chain acyl-CoA has an even number of carbon atoms, then the final products of the last cycle (i.e., the metabolism of a four-carbon acyl-CoA molecule) through the β-oxidation sequence are two acetyl-CoA molecules (Fig. 21.6b). However, if the last long-chain acyl-CoA has an odd number of carbon atoms (i.e., 5), then the products of the last cycle through β-oxidation are one acetyl-CoA and one propionyl CoA. Unlike acetyl-CoA, propionyl-CoA cannot enter the TCA cycle. However, propionyl-CoA is readily converted to succinyl-CoA, a TCA cycle intermediate (Fig. 21.6b). Hence, this is another pathway that contributes to the maintenance of the TCA cycle intermediate pool size. Both pyruvate molecules produced from glucose and odd-numbered fatty acid molecules that undergo complete β-oxidation contribute to maintenance of the TCA intermediate pool. Processes that add molecules to the TCA intermediate pool are termed anaplerotic (Fig. 21.6a, b), and those that remove intermediates from the pool are called cataplerotic. The importance of these processes to TCA cycle function will be illustrated in a clinical example of an inborn metabolic abnormality to be presented later.
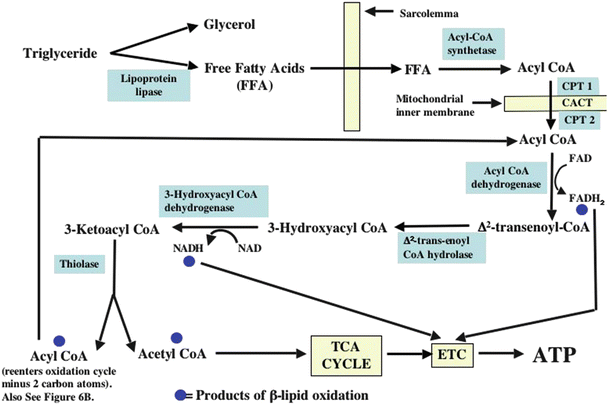
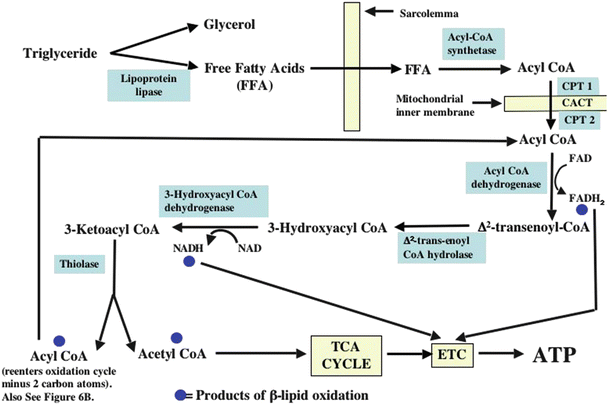
Fig. 21.12
Flowchart depicting the cellular uptake of free fatty acids and the pathways through which their metabolism proceeds. See text for discussion. ATP adenosine triphosphate, CPT 1 carnitine palmitoyltransferase 1, CPT 2 carnitine palmitoyltransferase 2, ETC electron transport chain, FADH 2 flavin adenine dinucleotide, FFA free fatty acids, NADH nicotinamide adenine dinucleotide, TCA tricarboxylic acid
The products of complete β-oxidation of a fatty acid are acetyl-CoA (and propionyl-CoA if the chain has an odd number of carbons), NADH, and flavin adenine dinucleotide (FADH2). However, consumption of these products by the TCA cycle and the electron transport chain cannot occur in the absence of oxygen. Thus, β-oxidation cannot occur under anoxic or ischemic conditions, and markedly ischemic myocardium does not support either pyruvate- or fatty acid-derived ATP synthesis. Hence, during ischemia, the only source of ATP synthesis is anaerobic glycolysis.
21.3.4 Regulation of Carbon Substrate Metabolic Pathways
Myocardial glycolysis is regulated at several levels (some of which are shown in Fig. 21.13a); the first is entry of glucose into the cell. In the heart and skeletal muscle, this process is largely dependent on the activity of the GLUT 4 glucose transporter. The quantity of the GLUT 4 transporter in the plasma membrane is determined by the action of insulin on the sarcolemmal insulin receptor, the activation of which begins a sequence of biochemical events that ultimately triggers migration of GLUT 4 molecules to the plasma membrane from cytoplasmic storage sites. Increased levels of fatty acid metabolites in cardiomyocytes inhibit insulin receptor activation and the consequent transport of GLUT 4 to the plasma membrane. Blood glucose levels are generally well defended by an organism, so glucose availability is not usually limiting to transport. Rather, the number of GLUT 4 molecules present in the plasma membrane usually determines the rate of glucose transport. In addition to insulin-associated increases, plasma membrane GLUT 4 levels are also enhanced during increased myocardial work states via the associated elevation of cytosolic adenosine monophosphate (AMP) and ADP. Increased AMP levels activate AMP-activated protein kinase (AMPK) , which causes increased GLUT 4 trafficking to the plasma membrane. The subsequent reactions of the glycolytic sequence (and glycogen breakdown) are also activated by a number of factors including increased cytosolic Ca++, AMPK, protein kinases A and C, PI3K, and fructose 2,6-bisphosphate, as well as AMP and ADP. These reactions are inhibited by increased levels of ATP and increased cytosolic levels of H+ and/or citrate and other factors as well. For example, increased free fatty acid metabolism increases mitochondrial citrate synthesis, and increased citrate exiting the mitochondria inhibits glycolysis. The first step of oxidative glucose metabolism (the decarboxylation of pyruvate to form acetyl-CoA) by PDH is also highly regulated by PDH kinases and phosphatases, and a high level of β-lipid oxidation causes inactivation of PDH by stimulating its phosphorylation by specific kinases. The regulatory pathways are complex and discussed in more detail in several references cited at the end of the chapter.
< div class='tao-gold-member'>
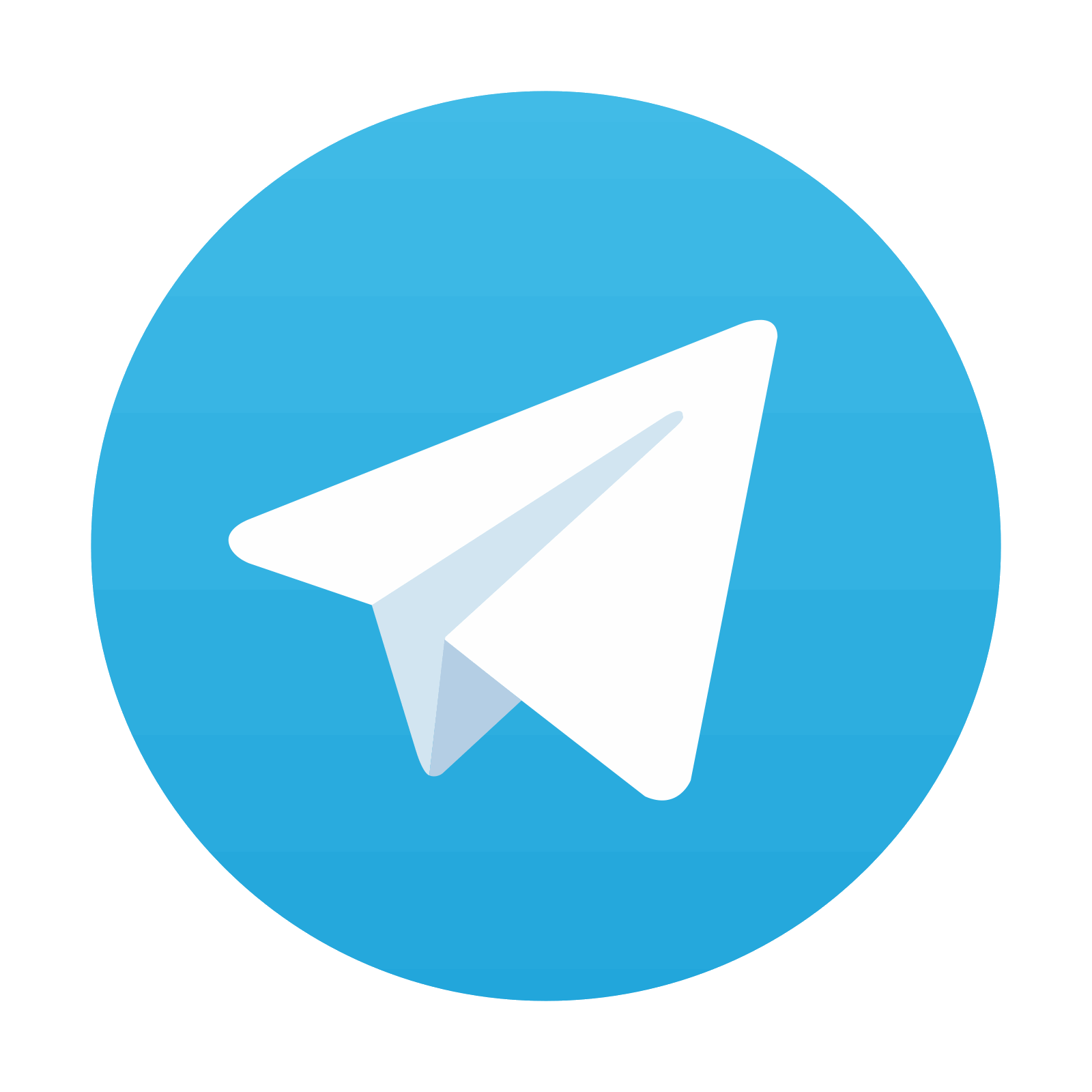
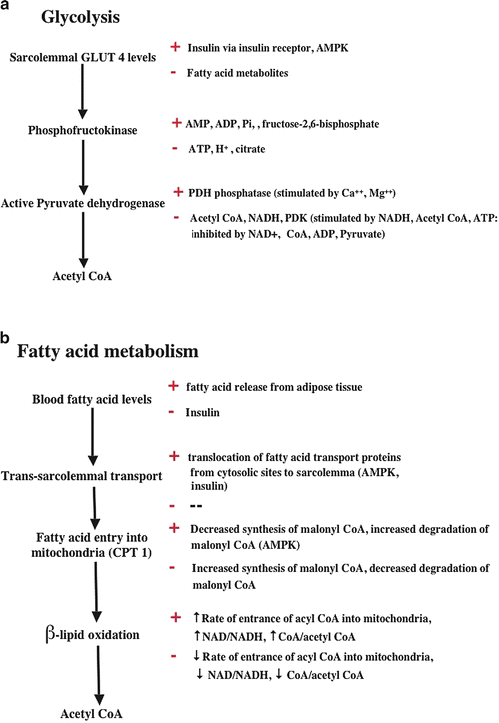
Only gold members can continue reading. Log In or Register a > to continue
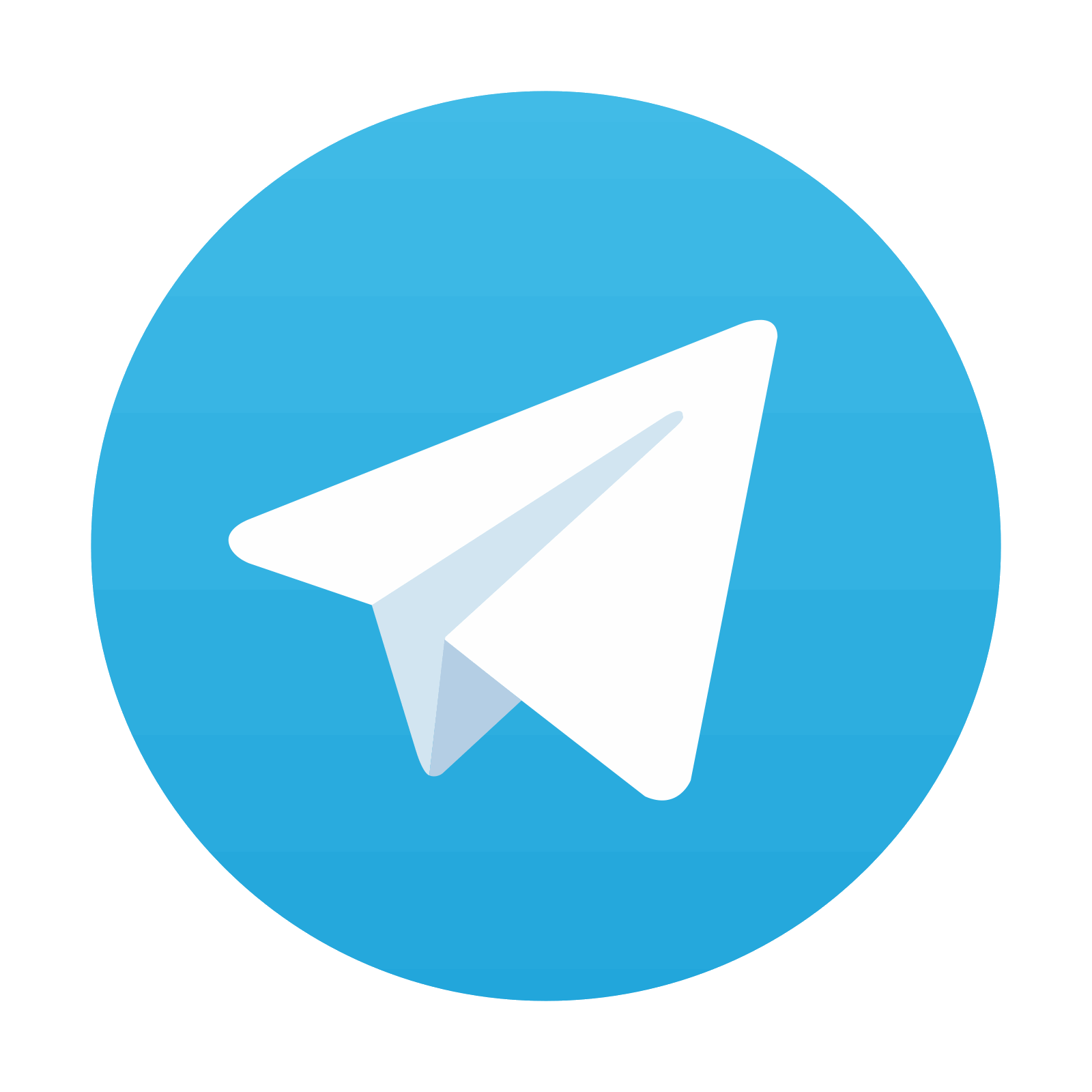
Stay updated, free articles. Join our Telegram channel
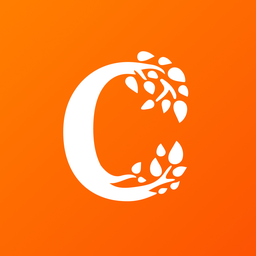
Full access? Get Clinical Tree
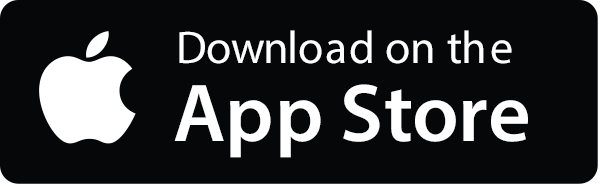
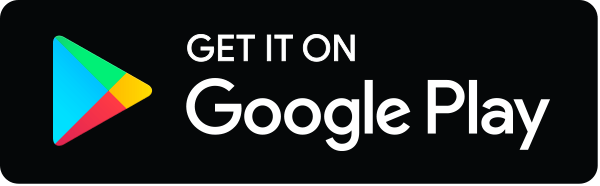