Fig. 1.1.
Scanning electron micrograph of C. albicans growing on oral epithelial cell layer, in yeast, hyphal and pseudohyphal morphologies. Hyphae invade via active penetration and induce endocytosis, indicated by membrane ruffling (Zakikhany K, Holland G, Özel M, Hube B, with permission)
In the commensal phase, C. albicans co-exists and interacts with bacteria of the microflora (Piispanen and Hogan 2012). These probiotic bacteria generally contribute to a balanced and protective immunity and can produce molecules such as fatty acids, thereby directly inhibiting fungal growth, blocking epithelial binding sites and competing for nutrients. Although nutrients are relatively rich in the gut, they are quickly absorbed by the microbial flora and epithelial cells, forcing colonising C. albicans cells to use nutrient acquisition mechanisms efficiently and to be metabolically flexible. Changing conditions (e.g. pH, osmolarity) in different host niches probably require stress adaptation mechanisms to be deployed for growth to be permissible. It also seems feasible that commensal microbes, such as C. albicans, have developed immune evasion strategies to avoid recognition because the trigger of immune responses or inflammation would cause additional stress. Nevertheless, occasionally invading hyphae may cause mild local damage, thereby attracting phagocytes. These transient confrontations with the immune system may select for fungal attributes that enable fungal cells to cope with situations beyond the commensal environment. This may include the refinement of transcriptional programs that prepare invading cells for challenges associated with the transition from commensalism to pathogenicity – a phenomenon that can be described as “predictive adaptation”. One of these programs is the transition from yeast to hyphal growth and the expression of hyphal-associated genes. These genes encode adhesins, invasins, iron acquisition factors, hydrolases, damaging factors and enzymes that neutralise reactive oxygen species.
In the invasive phase, fungal hyphae, not yeast cells, invade into and through epithelial cell layers, damage their membranes and exploit their cellular content as nutrients. At this stage, fungal cells are recognised, the immune system is activated and armed, and phagocytes (monocytes, macrophages, dendritic cells, neutrophils) are attracted, establishing a local battlefield. If the fungus succeeds and invades into deeper tissue or gains access to blood vessels via damaged barriers, fungal cells may enter the blood stream and escape through the endothelial layers to colonise the major organs, causing life-threatening systemic infections (Fig. 1.2). Attributes that enable the fungus to invade, cause damage, counteract the immune system, cope with changing stresses, exploit host molecules as nutrients, and colonise non-commensal niches are true pathogenicity attributes and are described in this chapter.
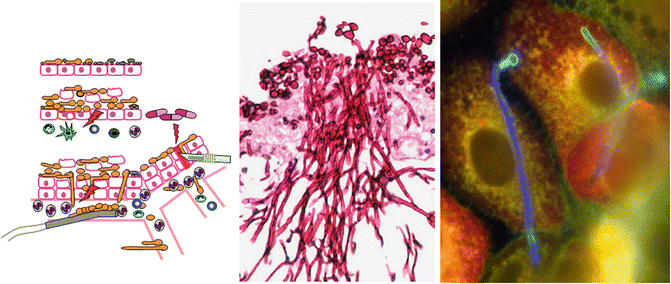
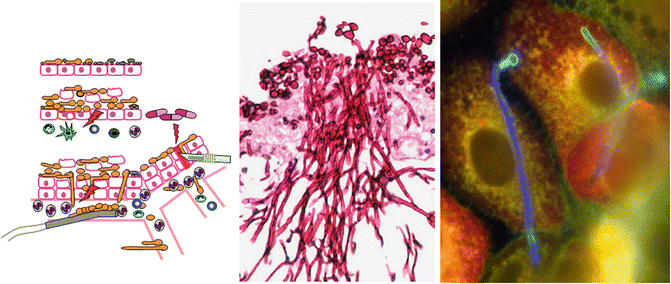
Fig. 1.2.
Left: Cartoon of the various states of C. albicans from commensalism on the epithelial surface to invasion and pathogenicity due to insult via immunosuppression, injury and introduction as a biofilm on foreign bodies. Middle: Invasive properties of hyphae of C. albicans on model epithelium from chick chorioallantoic membrane (Gow NAR, with permission). Right: C. albicans interepithelial invasion of hyphae through one oral epithelial cell and invasion into an adjacent cell. Dark blue parts of hyphae are intracellular and bright blue parts are extracellular (Almeida R, Hube B, with permission)
A. Nutrient Acquisition and Metabolic Flexibility
During the transition from commensal growth to pathogenicity, and during dissemination and colonisation of the different host organs and niches, the available local nutrients change dramatically. The fact that C. albicans can proliferate in all these niches indicates a high degree of metabolic flexibility and suggests that several host sources can be exploited as nutrients. During systemic infections, the fungus disseminates via the bloodstream, which is relatively rich in glucose (6–8 mM), the preferred carbon source of C. albicans. However, circulating phagocytes, in particular neutrophils and to a lesser extent monocytes, efficiently phagocytose C. albicans. Within the phagosome, the fungus is exposed to a nutrient-starved environment and switches from glycolysis to gluconeogenesis and activates the glyoxylate cycle. In fact, key enzymes of the glyoxylate cycle, such as isocitrate lyase and citrate synthase seem to be required for full virulence of C. albicans (Fradin et al. 2005; Lorenz and Fink 2002; Miramón et al. 2012). Single-cell profiling and global transcriptional profiling (Barelle et al. 2006; Thewes et al. 2007; Zakikhany et al. 2007) of hyphae invading epithelial tissues or organs showed that genes encoding for enzymes of both the glycolysis and glyoxylate cycles are activated, possibly reflecting different subpopulation of cells. Secreted lipases (Lips) and phospholipases B (PLBs) of C. albicans can degrade host lipids and phospholipids to provide non-glucose carbon sources. Secreted aspartic proteases (Saps) are able to hydrolyse host proteins, thereby releasing peptides and amino acids that can serve as a source of carbon and nitrogen. Indeed, C. albicans can use all natural amino acids as nitrogen sources (our unpublished data).
Interestingly, growth on carbon sources available within the host other than glucose, such as lactate or amino acids, can render C. albicans more resistant to environmental stresses and can increase its virulence potential in vivo (Ene et al. 2012). A combination of carbon sources, or alternatives to glucose and fructose such as pyruvate, sorbitol, oleic acids and galactose, increase resistance to osmotic stress. With the exception of oleic acid, this corresponds to an increase in kidney burden in systemically infected mice (Ene et al. 2012). However, the impact of carbon source on virulence depends on the site of infection in mouse models, e.g. cells grown on oleic acid produce greater vaginal infection and low systemic infection, although systemic infection is enhanced by growth on lactate and amino acids (Ene et al. 2012). Additionally, unlike the non-pathogenic Saccharomyces cerevisiae, C. albicans is able to assimilate lactate and oleic acid in the presence of glucose (Sandai et al. 2012), allowing C. albicans to swiftly adapt to an environment in which nutrient availability can change as quickly as a phagocyte can engulf a cell. This metabolic flexibility and the consequent protective benefits under stress give C. albicans an advantage within various host environments, under either commensal or pathogenic conditions.
B. Micronutrients
Iron is an essential element for both the host and C. albicans. However, in the host, iron is almost completely associated with host proteins, which prevents not only iron-dependent production of toxic free radicals, but also microbial growth (“nutritional immunity”). Like other commensals and many other pathogens, C. albicans has developed several strategies for iron acquisition within the host (Almeida et al. 2009). Although C. albicans cannot produce siderophores, the fungus can exploit iron from siderophores produced by other microbes, an iron acquisition strategy that may be particularly useful in the commensal stage. Siderophores and other iron complexes are taken up by the Sit1/Arn1 transporter (Heymann et al. 2002; Hu et al. 2002).
In addition to uptake of siderophores, C. albicans can exploit iron from iron-containing host proteins. For example, haemoglobin can be used as an iron source during the pathogenic stage. Haemoglobin iron utilisation may occur predominantly via hyphae, which can bind to erythrocytes (Moors et al. 1992). Following the release of haemoglobin by an unknown mechanism, specific receptors on the surface of C. albicans such as RBT5, RBT51, WAP1/CSA1, CSA2 and PGA7 mediate uptake (Weissman and Kornitzer 2004). This process also seems to be hyphal-associated because the expression of some haemoglobin receptors is co-regulated with hyphal formation (Braun et al. 2000). Once haemoglobin is internalised, it is hydrolysed or denatured and haem is released (Weissman et al. 2008). A haem oxygenase (Santos et al. 2003), encoded by HXM1, degrades haem intracellularly (Pendrak et al. 2004). Another host molecule that can serve as an iron source for C. albicans, at least in vitro, is transferrin. Although the transferrin receptor remains unknown, it was shown that binding is necessary for iron utilisation from transferrin (Knight et al. 2005). Furthermore, C. albicans is able to bind the main host iron storage protein ferritin on the surface of hyphae via the hyphal-associated adhesin and invasin Als (see below) (Almeida et al. 2008). Following binding, the iron content of ferritin must be released by a mechanism that probably includes acidification. In fact, C. albicans is only able to use ferritin as an iron source under conditions that permit acid production, suggesting that iron acquisition from ferritin by C. albicans is pH-mediated (Almeida et al. 2008).
To utilise iron from transferrin, from ferritin or from the environment, C. albicans uses the reductive pathway, which consists of several ferric reductases, multicopper oxidase(s) and iron permeases. The extracellular pH greatly influences the availability of iron because neutral and alkaline pH favours the oxidisation from the soluble Fe2+ to the non-soluble Fe3+ ion. The RIM101 pathway of C. albicans is required for an appropriate transcriptional response to the environmental pH by coordinating the upregulation of genes involved in the reductive pathway, for example by upregulation of ferric reductases and the ferritin receptor Als3 under alkaline conditions (Baek et al. 2008; Liang et al. 2009; Nobile et al. 2008).
In addition to iron, further trace metals such as zinc, manganese and copper are also essential for growth and survival of C. albicans in the commensal and pathogenic phases, suggesting that nutritional immunity is a much more extensive phenomenon than iron sequestration. Yet, the mechanisms of acquisition of metals other than iron are largely unknown. A novel mechanism for zinc acquisition by C. albicans was recently described (Citiulo et al. 2012) by which the fungus secretes the zinc-binding pH-regulated antigen 1 (Pra1). This binds extracellular zinc and re-associates with a Pra1 receptor and zinc transporter (Zrt1) on the cell surface. Because this system resembles iron uptake via siderophores, Pra1 was named a “zincophore”.
III. Stress Response
Whether in the pathogenic phase or commensal phase of interaction with the host, C. albicans faces a number of environmental and immune-derived stresses. Each niche with which the fungus is associated presents unique environmental factors, from the varying pH of the gastrointestinal and urogenital tracts (Brown et al. 2012) to the osmotic pressures of the oral mucosa and kidneys. A multifaceted and robust stress response is crucial for commensal adaptation, but also for virulence (Brown et al. 2012).
Many environmental and stress signals in fungi are sensed via mitogen-activated protein kinase (MAPK) pathways. As with numerous classical signalling pathways, particularly MAPK pathways, signals are propagated as a series of phosphorylation events, each kinase phosphorylating the next in turn until transcription factors are activated. Three such MAPK pathways are activated in C. albicans, with each pathway distinguished by a specific kinase, Mkc1, Hog1 or Cek1 (Monge et al. 2006).
The Hog1 MAPK pathway is considered a core response and is activated after many types of stresses (Monge et al. 2006), including oxidative and osmotic stresses. Hog1 also plays a role in morphogenesis, cell wall formation and the response to thermal stress (Monge et al. 2006). However, Hog1 phosphorylation occurs at different times post stress in response to osmotic, oxidative and nitrosative stresses, even responding to osmotic stress from sodium chloride and sorbitol at distinct time points (Kaloriti et al 2012). Activation of Hog1 in response to osmotic stress leads to accumulation of glycerol within the cell, which counters the loss of water due to chemical gradients (Monge et al. 2006). Also responding to oxidative and osmotic stress, the Mkc1 pathway is primarily noted for cell wall salvage and biogenesis as well as for maintaining cellular integrity (Monge et al. 2006; Munro et al. 2007), whereas the Cek1 pathway mediates filamentation and mating, and possibly responds to quorum sensing molecules (Mayer et al. 2012; Monge et al. 2006). Cells without Cek1 are unable to form hyphae in low-nitrogen media, although this can be overcome by the presence of serum (Monge et al. 2006). As would be anticipated, the ability to respond to the host battery of stresses is crucial for the success of C. albicans as a pathogen because deletion of the major stress response components Mkc1, Hog1 or Cek1 results in attenuated virulence in the mouse model (Alonso-Monge et al. 1999; Csank et al. 1998; Diez-Orejas et al. 1997).
Within the host, further environmental stressors are also produced by the immune system, specifically phagocytic cells capable of producing reactive oxygen species (ROS) and reactive nitrogen species (RNS). In its role as a pathogen, C. albicans copes with both of these stresses with a series of detoxifying enzymes. The oxidative stress response to ROS such as hydroxyl radicals and peroxide includes synthesis of antioxidants, detoxifying enzymes such as catalase (Cta1) and superoxide dismutases (Sod1-5), and repair of damaged proteins (Brown et al. 2012; Hwang et al. 2002; Martchenko et al. 2004; Wysong et al. 1998). C. albicans cells phagocytosed by neutrophils are exposed to RNS, which may be detoxified by flavohaemoglobin enzyme Yhb1 (Hromatka et al. 2005; Tillmann et al. 2011; Miramón et al. 2012). Loss or deletion of some of the enzymes that detoxify ROS and RNS impacts virulence in animal models (Hromatka et al. 2005; Hwang et al. 2002; Martchenko et al. 2004; Tillmann et al. 2011; Wysong et al. 1998).
Although much work has been done on responses to osmotic, oxidative and nitrosative stresses singly, within the host – and particularly when in contact with host phagocytic cells – it is usual for several stresses to confront C. albicans simultaneously. It has been found that a combination of stresses similar to those encountered in vivo by C. albicans can efficiently kill the fungus, despite intact stress response pathways (Kaloriti et al. 2012). The activation of the individual stress response and salvage pathways under combinatorial stress has not yet been described.
C. albicans shows a great deal of flexibility when confronted with the broad range of pH found in host tissues. Under conditions of acidic pH and in the presence of exogenous amino acids, C. albicans is able to rapidly raise extracellular pH through export of ammonia, auto-inducing filamentation (Vylkova et al. 2011). Alkaline pH is a known inducer of filamentation in C. albicans, and this is dependent upon Rim101. Cells deprived of Rim101 are unable to form hyphae in alkaline pH (Davis et al. 2000). Both the ability to filament in alkaline pH and the alkalinisation of extracellular spaces provide the fungus with the means to escape from phagolysosomes.
Heat shock proteins (Hsp) prevent protein unfolding and aggregation by acting as chaperones, and the heat shock response is a conserved response to high temperature and oxidative stress (Lindquist 1992). Currently, six major and six small heat shock proteins have been identified in C. albicans, with functions in thermal tolerance, virulence, drug resistance and biofilm formation (Mayer et al. 2013). Hsp90 facilitates thermal tolerance via the Hog1, Cek1 and Mkc1 pathways (Leach et al. 2012) and regulates temperature-dependent morphological changes (Shapiro and Cowen 2010). The small heat shock protein Hsp21 mediates both stress adaptation and virulence via phosphorylation of Cek1 (Mayer et al. 2012). Heat stress also results in accumulation of trehalose, the function of which has not been fully elucidated (Brown et al. 2012).
IV. Structure and Function of the Cell Wall in the Host
The cell wall of C. albicans is composed almost exclusively of molecules that are absent from the human body and is therefore a key target for immune recognition (Netea et al. 2008; Romani 2011). The cell wall is also essential for viability and one sixth of the total genome is invested in its biosynthesis and maintenance. The cell wall is highly dynamic and the changing nature of the cell surface presents challenges for the detection and surveillance of the fungus. This is important in terms of commensalism and pathogenicity because it is the molecules of the cell wall that activate immune recognition. Over-activation of pro-inflammatory immune responses can be a major cause of tissue damage.
The wall exists in two readily distinguished layers: an outer layer that is enriched with highly glycosylated glycoproteins, many of which are expressed in a morphology-dependent fashion. Many proteins are found predominantly in the yeast or hyphal growth phase and hyphae-specific cell wall proteins play roles as adhesins and invasins and in modulating the immune responses (Liu and Filler 2011; Luo et al. 2010). There is less mannan and substantially more chitin in the hyphal wall. Cell wall mannan comprises short O-linked linear α-1,2 mannan; highly branched N-mannan comprises an α-1,6 mannan backbone and side chains composed of α-1,2 mannose and α-1,3 mannose residues, with β-1,2 mannose attached to the acid-stable and phosphodiester acid-labile side chains. Both N-mannan and O-mannan may also contain phosphomannosylated side chains. Mannan chemistry defines many of the Candida serotypes, further underlying the heterogeneity that exists in mannan structure (Suzuki 2002). C. albicans O-mannans consist of 1–5 mannose sugars that are almost exclusively α-1,2 linked. The phosphomannan is exclusively β-1,2 mannose, which is a linkage that is not present in many other yeast species.
The inner cell wall represents the skeletal layer and is composed predominantly of two robust polysaccharides, β-1,3 glucan and lesser amounts of chitin, that confer strength and cell shape (Fig. 1.1). In budding yeast cells, a scar is left on the mother cell after separation of the bud and, at this site, components that constitute the inner layers of the cell wall, such as chitin and β-1,3 glucan, may become exposed at the surface (Gantner et al. 2005).
Therefore, although the basic components of the cell wall of C. albicans are similar in its yeast and filamentous forms, the signature of proteins and amounts of individual pathogen-associated molecular patterns (PAMPs) presented to immune cells differs substantially in these different cell types. In addition, the carbon source for growth (see above) and the growth conditions result in substantial remodelling of the cell wall, even if the morphology of the cell is not affected (Gow et al. 2002).
V. Morphology
The three major tissue-associated morphologies of C. albicans are the spheroid yeast cell and the broadly filamentous hyphae and pseudohyphae (Fig. 1.1) (Sudbery et al. 2004). Although the strict function of each morphological form within the host is up for debate, yeast cells are presumed to disseminate more readily through the host whereas hyphae exhibit more invasive tendencies (Gow et al. 2002; Sudbery et al. 2004). However, it must be kept in mind that yeast cells are the invasive form in other fungal pathogens – including all those dimorphic fungi that have an environmental hyphal phase. Less well characterised is the impact of the pseudohyphal form on the ability of C. albicans to cause disease or establish a commensal state within the host. Certainly, all three forms (yeast, pseudohyphal and hyphal) are found in clinical specimens as well as in animal models of infection.
Although it is clear that the majority of clinically relevant Candida species predominate in the yeast form (Thompson et al. 2011), the specific ability of C. albicans to form both yeast and hyphal cells in vivo seems to be an important attribute in the repertoire of virulence factors in this organism. The capacity to undergo reversible yeast–hyphae morphogenesis is linked to the virulence of C. albicans (Gow et al. 2002; Lo et al. 1997; Sudbery 2011). A wide range of studies have shown that mutants that are affected in the mechanism that regulates the yeast–hyphae and hyphae–yeast transition are less virulent in animal models than wild-type C. albicans strains (Lo et al. 1997; Saville et al. 2003). The dependence on the ability to switch to the hyphal form for virulence is evident in every commonly used animal model of C. albicans infection, both vertebrate and invertebrate (Brothers et al. 2011; Davis et al. 2011; Lo et al. 1997; Pukkila-Worley et al. 2009).
To date, numerous genes involved in the morphological switch from yeast to hyphae have been characterised and studied in detail, though very few are noted for their contribution to commensalism. The expression and activity of transcription factors Efg1 and Efh1, in particular, are considered modulators of the commensal state within the gut (Pierce and Kumamoto 2012; Pierce et al. 2013; White et al. 2007). It was found that when colonising the gut of mice, C. albicans remained primarily in the yeast form (White et al. 2007), and that the absence of Efg1 promoted high early colonisation in healthy and athymic mice (Pierce and Kumamoto 2012). It may therefore be inferred that C. albicans with low Efg1 levels are the most likely commensal form, even in immunocompromised hosts, unless and until an environmental shift promotes more pathogenic phenotypes.
Specimens from humans and experimental animals show that C. albicans hyphae dominate at the primary site of infiltration of epithelial cell layers and tissues, whereas yeast cells are generally found either on the epithelial cell surface or emerging from penetrating hyphae that are infiltrating tissue (Fig. 1.2, middle) (Ray and Payne 1988; Scherwitz 1982). Furthermore, it is proposed that there is a threshold for the amount of C. albicans that is tolerated. Therefore, the host immune defences have to keep the fungal burden below the threshold and have to distinguish a non-pathogenic C. albicans from an invasive and potentially life-threatening C. albicans in order to keep homeostasis (Moyes et al. 2010).
As detailed below, much recent progress has been made on the pattern recognition of C. albicans. However, much less is known regarding the differential recognition between the two main morphogenetic forms of C. albicans, yeasts and hyphae. Because this fungus reversibly transits between these forms during the progression of an infection, yeast–hyphal morphogenesis is likely to have a major effect on spatial and temporal aspects of the immune response.
VI. Adhesion, Invasion and Damage: Interaction with Epithelial Cells
Attachment of C. albicans to its host is a property that is required during both the commensal and the pathogenic phase. Adhesion is mediated by physical surface attributes such as hydrophobicity and specialised surface proteins, the adhesins. These proteins mediate adhesion to host cells, other Candida cells, other microbes or abiotic surfaces (e.g. catheters). The large number and variety of adhesins enables C. albicans to colonise several host niches. Adhesins are often glycosylphosphatidylinositol (GPI)-proteins or GPI-anchored proteins, which are exposed at the cell surface (Zordan and Cormack 2012). One of the best-studied group of C. albicans adhesins are the agglutinin-like sequence (Als) proteins, which form a family consisting of eight GPI-linked glycoproteins (Als1–Als7 and Als9) (Hoyer 2001). Each Als protein has three characteristic domains: a conserved N-terminal domain, a central domain with short tandemly repeated copies and variable length, and a C-terminal domain that varies in both sequence and length.
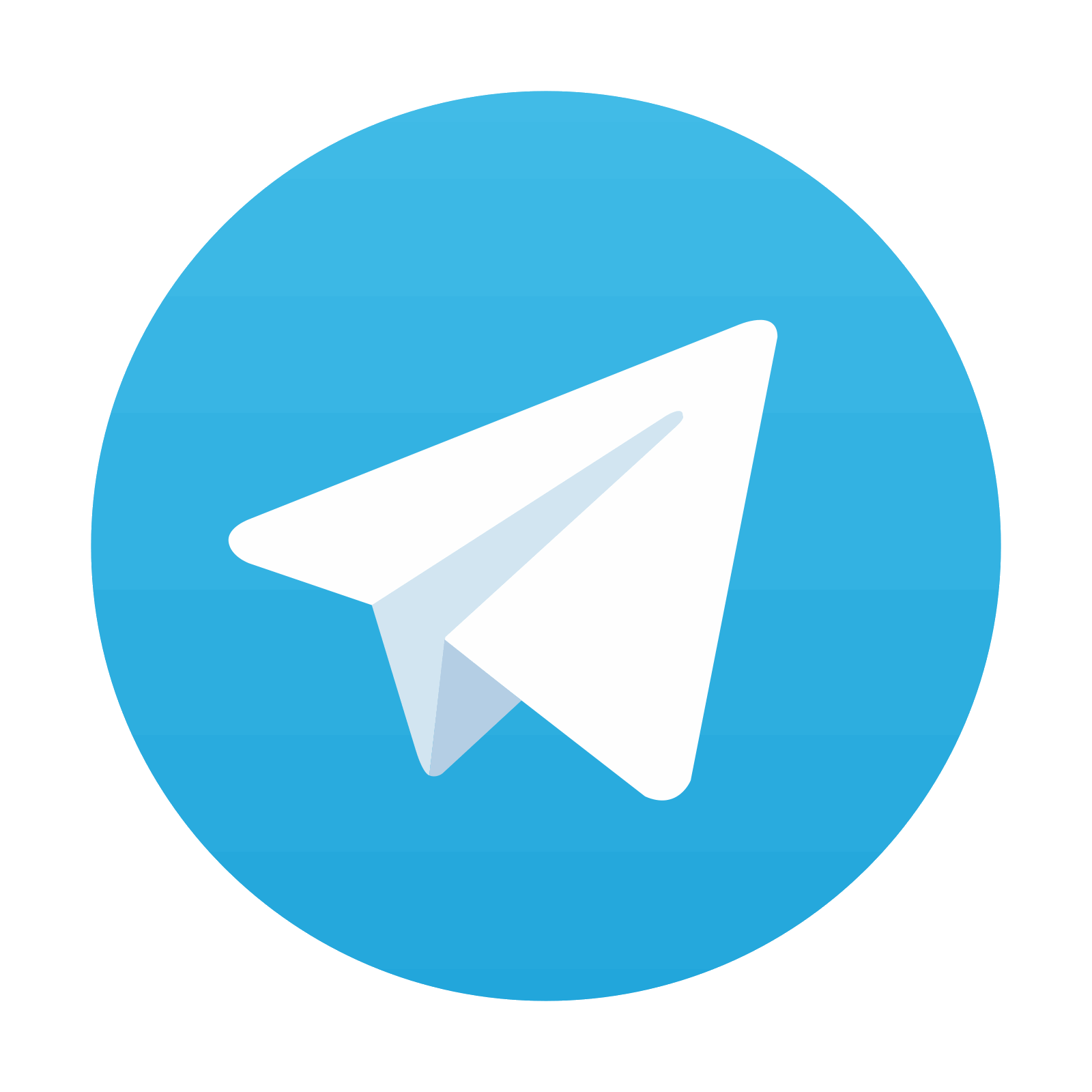
Stay updated, free articles. Join our Telegram channel
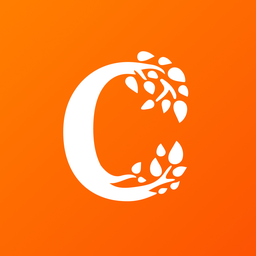
Full access? Get Clinical Tree
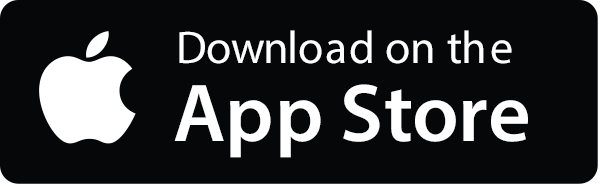
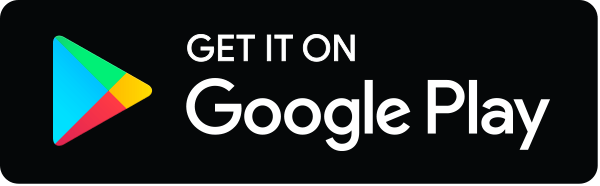