Introduction
Extracorporeal membrane oxygenation (ECMO) is a technique of life support that consists of diverting a fraction of the patient’s blood flow (BF) through an artificial lung for gas exchange (oxygenation and carbon dioxide [CO 2 ] removal) and then returning it to the patient. Thanks to improvement in technology and materials and to a deeper understanding of pathophysiology, the indications, timing, and management of ECMO have changed substantially over the years. This chapter introduces the concept of ECMO and discusses its use for respiratory support in patients with acute respiratory failure (ARF).
Principles of ECMO
ECMO is a generic term used to describe a number of different techniques used for prolonged artificial cardiac and/or respiratory support. Depending on the returning vessel (venous or arterial), ECMO can be used for cardiac ( veno-arterial bypass [VA-ECMO]) or respiratory support. For respiratory support, blood can be drained either from a vein ( veno-venous ECMO [VV-ECMO]) or from an artery ( arterio-venous ECMO [AV-ECMO]). During VA- and VV-ECMO in which blood is drained from a vein, the blood is withdrawn with the action of a pump, whereas during AV-ECMO, the arterial pressure is the driving force. BF passes through the oxygenator ( membrane lung [ML]), where oxygen is added and CO 2 removed. The efficiency in CO 2 removal is significantly higher than for oxygenation. Depending on the BF through the circuit, the technique may be used mainly for CO 2 removal (BF < 2 L/min) or for both CO 2 removal and oxygenation (BF up to 5 to 6 L/min). For an appreciation of the different techniques available for respiratory support, it is useful to provide a brief historical perspective.
ECMO Indications and Technology: a Historical Perspective
The birth of ECMO dates back to 1939 with the development of the first heart-lung machine by John Gibbon; this culminated in 1953 with the first open heart operation. The first successful use of prolonged ECMO was reported by Hill and colleagues in 1972 in a 22-year-old patient with acute respiratory distress syndrome (ARDS). In the following 2 years, other successful cases were reported. Due to the extremely high mortality rate of ARDS patients at the time, these first reports evoked great interest and were the impetus for the first multicenter randomized clinical trial of prolonged ECMO for adults with ARDS. The study, commissioned by the National Institutes of Health, failed to show any survival benefit from ECMO. The reasons for failure of this trial were not clear, but the negative results led to a virtual cessation of laboratory and clinical research on adult ECMO. Fortunately, a number of determined investigators continued studying and improving the technique. Currently, there is a resurgence of interest in ECMO and its clinical application.
Important factors contributing to the maintenance of the interest in ECMO were the encouraging results reported in neonatal respiratory failure. The first successful treatment in newborns was reported by Bartlett and colleagues in 1976, followed in the subsequent few years with several encouraging case series from different groups. Two prospective randomized controlled studies were then performed: the first by Bartlett’s group in Michigan in 1985, the second by O’Rourke and coworkers in Boston in 1989. Both studies were positive and in a few years, ECMO became standard treatment for neonatal respiratory failure.
In adults, major advances came from the pioneering work of Kolobow and Gattinoni, who focused on why the NIH-ECMO trial had failed. At the time, the aim of ECMO was to provide reasonably normal blood gases, essentially buying time for the lungs to heal. As such, high ECMO BF rates were necessary to improve oxygenation, and the veno-arterial configuration was the standard. In 1977, recognizing that oxygenation and carbon dioxide elimination were based on different physiologic mechanisms, Gattinoni and Kolobow realized that by removing CO 2 through the membrane lung, ventilation of the native lung (NL) could be reduced virtually to zero. They showed that nearly all the metabolic CO 2 production could be removed through an artificial lung using much lower extracorporeal BFs than those required to oxygenate the blood. On the basis of these observations, in 1979, they developed the concept of extracorporeal CO 2 removal (EC co 2 R), proposing the use of a VV bypass configuration instead of the classical VA mode and the use of low-frequency intermittent positive pressure ventilation (LFPPV) to allow the lung to rest. In 1980 Gattinoni and colleagues reported the successful clinical application of this technique, and in 1986 they published the results of the first study on EC co 2 R-LFPPV in 43 patients with severe ARF. Unfortunately, these results were not confirmed by a subsequent single-center, randomized controlled trial conducted by Morris and colleagues in 40 ARDS patients. This second failure led to an almost complete stop of ECMO development in adults, confining its application to newborns. Nevertheless, a few centers around the world continued to apply ECMO in adult patients, with important technical improvements.
At least five reasons have led to the recent resurgence of interest in ECMO:
- 1.
Technical developments. There have been a number of technologic advances that have decreased the complications associated with the earlier application of ECMO. Oxygenators, propelling pumps, and cannulas have all undergone substantial improvements. The available equipment now allows easier, safer, and longer ECMO application. An important factor has been the miniaturization of the whole system, making it possible to use ECMO for interhospital transportation.
- 2.
The Extracorporeal Life Support Organization (ELSO). Founded in 1989, ELSO includes most ECMO centers around the world. An important function of ELSO is to maintain a registry of ECMO cases and to provide annual reports provide, which information on ECMO usage, survival rates, and complication rates. ELSO has also developed guidelines that represent the most important reference for ECMO users worldwide.
- 3.
The concept of ventilator-induced lung injury (VILI) and improvement in ARDS treatment. Recognition that mechanical ventilation, though necessary to preserve life, can exacerbate lung damage eventually contributing to mortality, probably represents the most important advance in ARDS research. Ventilation with high tidal volumes (V t ) and high distending pressures has been recognized as a major cause of VILI. In the seminal ARDS-Network randomized controlled trial, patients ventilated with a V t of 6 mL/kg of predicted body weight (PBW) had a significantly lower mortality than those ventilated with a V t of 12 mL/kg PBW. These findings led to the concept of “protective ventilatory strategy,” largely consisting of low V t (6 to 8 mL/kg PBW) and limitation of plateau airway pressures (Pplat < 28 to 30 cm H 2 O). It is important to understand that the concept of VILI was not recognized early in the history of ECMO; for this reason, in the two aforementioned ECMO randomized trials, no attention was paid to V t and airway pressures in either treatment group.
- 4.
The Conventional Ventilation or ECMO for Severe Adult Respiratory Failure (CESAR) trial. The CESAR trial, conducted in the United Kingdom and published in 2009, was the first randomized clinical trial showing a survival advantage of ECMO in adults. Adult patients (18 to 65 years) with severe but potentially reversible ARF (defined as a Murray score ≥3 or uncompensated hypercapnia with a pH < 7.20) were enrolled. Patients randomized to receive ECMO were transferred to the Leicester ECMO center, while controls remained in designated treatment centers. Between 2001 and 2006, 180 patients were enrolled. Survival or absence of severe disability at 6 months was 63% in the ECMO group, comparing favorably with 47% in the control group; the authors concluded that referral of severely hypoxemic ARDS patients to a specialized center able to provide ECMO may increase survival. Two major limitations of the study have been identified. First, not all patients allocated to the ECMO group received ECMO because they died before or during transportation (5 patients) or they improved with conventional treatment after transportation to the ECMO center (17 patients). Second, there was no standardized protocol for mechanical ventilation in the control group, resulting in significantly fewer patients receiving a protective ventilatory strategy. As such, a criticism was that the patients randomized to ECMO were treated in a single specialized center, possibly receiving better care. However, despite these limitations, the results of the study have led to an increase in interest in ECMO worldwide.
- 5.
The H1N1 influenza outbreak. During the 2009 Influenza A (H1N1) pandemic in Australia and New Zealand, 61 patients with H1N1-associated ARDS received ECMO for refractory hypoxemia: survival was 78%. Following the Australian experience, several countries in the northern hemisphere prepared for the pandemic and case series were published reporting survival rates ranging from 68% to 83%. The British ECMO group recently published a cohort study in which patients with H1N1-associated ARDS referred for ECMO to one of the four adult ECMO centers in the United Kingdom were matched with similar non-ECMO treated patients using data from a concurrent, longitudinal cohort study. Hospital mortality rate of ECMO-referred patients was almost one half of that of non–ECMO-referred patients with all the three matching methods used.
Indications for Veno-Venous and Arterio-Venous ECMO
The primary indication for VV-ECMO is hypoxemic respiratory failure in patients with a high risk of mortality. According to ELSO guidelines, ECMO is indicated in patients with arterial P o 2 (Pa o 2 )/F io 2 less than 80 mm Hg with F io 2 greater than 90% and a Murray score of 3 to 4. Although the evidence backing up this recommendation is weak, similar indications have been suggested by different groups as, for example, in relation to H1N1 patients. An important finding, described by several investigators is the association between increased mortality and more days of mechanical ventilation before ECMO institution. This observation brings to mind the concept of VILI and suggests the importance of starting ECMO before lung injury has become irreversible. In the past few years, several case reports have been published on the use of VV-ECMO for non-ARDS indications, such as ARF due to severe trauma, pulmonary embolism, severe asthma, and as a bridge to lung transplant.
In comparison, the primary indication for AV-ECMO is hypercarbia in patients with respiratory failure and adequate cardiac function. Because the flow through the membrane lung is from the arterial blood, the ability to oxygenate the blood is less than if the inflow was venous blood; instead, the main value of AV-ECMO is the ability to remove CO 2 . AV-ECMO is discussed later in “Low-Flow CO 2 Removal: Indications and Technology,” under “Arterio-venous CO 2 removal.”
Contraindications to ECMO include advanced age, severe disability, and incurable malignancy. Uncontrolled coagulopathy, major bleeding, and prolonged mechanical ventilation are considered relative contraindications. The decision to start ECMO is made on a case-by-case basis.
Materials Necessary to Implement Veno-Venous ECMO
Basic components necessary for VV-ECMO are the cannulas for vascular access, a pump to propel the blood, and a gas exchange unit ( Fig. 103-1 ). Compared with the systems commonly used during cardiac surgery, ECMO systems are simpler. A schematic of the minimal requirements for a VV-ECMO circuit is shown in Figure 103-2 .


Oxygenators
Hollow-fiber oxygenators with a polymethylpentene membrane have become the standard for long-term treatment. Compared with the original silicon membrane, hollow-fiber oxygenators have a smaller priming volume, higher gas transfer rates, and much lower resistance. The use of polymethylpentene has also completely resolved the problem of plasma leakage that represented the main limitation to the use and diffusion of polypropylene hollow-fiber oxygenators. The gas and blood compartments are channeled inside and outside the hollow fibers, respectively. A third compartment for heat exchange is commonly present. Each compartment has an input and an output port.
Pumps
Similar to the diffusion of low-resistance, hollow-fiber oxygenators, centrifugal pumps have almost completely replaced roller pumps for long-term applications. Modern centrifugal pumps have a hole in the center of the rotor (Mendler design) and use a magnetically suspended and driven pump that eliminates stagnation, thrombosis, and heat production, which were common complications of earlier centrifugal pump models.
Cannulas
Vascular cannulas for ECMO have a thin wall, commonly made of polyurethane, and are often reinforced with wire to prevent kinking or collapse. Venous cannulas have side holes close to the tip or along the last 15 to 20 cm (multistage cannulas) to facilitate drainage of blood from the venous system. Modern cannulas have heparin-coated surfaces to increase biocompatibility and reduce activation of the clotting cascade. Recently, some double-lumen cannulas have become available. The most popular is the Avalon Elite designed by Wang and Zwischenberger. With this cannula, blood is drained from both the inferior and superior vena cava and returned directly into the right atrium. VV-ECMO with a single double-lumen cannula approach is gaining popularity over the traditional configuration with two cannulas.
Tubing
The tubing used for ECMO is made of polyvinylchloride, polyurethane, or silicon rubber. Appropriate connectors, with side ports for standard stopcocks, allow connection between different circuit components. All tubing and connectors surfaces can be coated with heparin and biocompatibility linings to reduce the risk and intensity of the thrombotic and inflammatory reactions triggered by the contact between blood and the artificial surfaces.
Cannulation for Veno-Venous ECMO
The technique of choice for VV-ECMO cannulation is the percutaneous approach. This technique, introduced in the late 1980s, has essentially become the standard of care. The main advantages are reduced risk of bleeding, shorter operative time, and easier mobilization and nursing of the patient.
Choice of cannula size and positioning is crucial. As a general rule, the size of the cannula should not exceed two thirds of the vessel diameter. Drainage cannulas should be big enough to ensure adequate flow with relatively low suction pressure, and the holes and tip of the cannula should be positioned in a high-flow vessel. Common sizes for drainage cannulas in adults range from 21 to 28 French Positioning is important to maximize flow and minimize recirculation. Recirculation is the shunting of the returned, oxygenated blood back into the intake for the ECMO. Recirculation will reduce the efficiency of ECMO and also increase the oxygenation of the intake blood so that it no longer reflects the true mixed venous oxygen saturation.
Veno-venous bypass may be accomplished using three different configurations: femoral-jugular, jugular-femoral, and femoral-femoral. Factors to consider when choosing the configuration are the drainage capability, recirculation risk, patient mobilization, and complications. The femoral-jugular access is the more frequently used; with a correctly positioned femoral cannula of 23 to 25 French, BF rates up to 6 to 7 L/min are easily obtained with minimal recirculation. The jugular-femoral approach, in which blood is drained directly from the right atrium, likely provides the best drainage but there is a high degree of recirculation, which can effectively nullify the drainage advantage. The femoral-femoral approach offers safer access with less possibility of accidental cannula dislocation; mobilization of the patient’s head is facilitated at the expense of lower limb mobilization. With this approach, however, minimization of recirculation requires careful positioning of the cannula tips, which is generally more difficult to obtain than with other approaches. The tip of the femoral drainage cannula should be positioned in the inferior vena cava superior to the renal veins, approximately at the level of L1-L2 lumbar vertebras.
Double-lumen cannulas are commonly placed through the right internal jugular vein. To obtain blood flow rates comparable with those commonly achieved with the traditional two-cannula configurations, insertion of cannulas up to 31 to 35 French may be necessary.
Choice of the cannulas is also based on the clinical indication. For more severely hypoxemic patients, larger cannula sizes and configurations allowing higher blood flows should be selected from the beginning. Insertion of a second drainage cannula, an upgrade from a single- to a double-cannula configuration, or a switch from VV- to VA-ECMO is sometimes mandated by changes in the patient’s needs.
Patient-Machine Interaction during Veno-Venous ECMO
The effect of VV-ECMO on arterial blood gas values is a function of the complex interplay among different factors. It is useful to discuss the effects on oxygenation and on CO 2 removal separately.
Effect of Veno-Venous ECMO on Oxygenation
During VV-ECMO, the membrane lung and native lung are in series ( Fig. 103-3 ). We can understand the physiology of VV-ECMO by following the changes in blood O 2 content from the vena cava (Cvc o 2 ) to the arterial side (Ca o 2 ). Cvc o 2 is a function of Ca o 2 , cardiac output (CO), and arterial-venous O 2 difference (Ca o 2 − Cvc o 2 ). A fraction of the venous return (BF/CO) is diverted through the membrane lung. The oxygen delivery of the membrane lung is given by = BF × (C o 2out − C o 2in ), where C o 2in and C o 2out are the oxygen content of the blood, respectively, entering (input) and leaving (output) the membrane lung. The oxygen content of the blood returning to the lung (the mixed venous blood,
) is the flow-weighted average of C o 2out and Cvc o 2 :
= (C o 2out × BF + Cvc o 2 × [CO-BF])/CO. In practice, the resulting effect of the membrane lung is to increase the O 2 content of the blood returning to the lung from Cvc o 2 to
.
is then increased to Ca o 2 by the residual oxygenating capacity of the patient’s lung. The oxygen delivery of the native lung is
= CO × (Ca o 2 −
), and the patient’s total oxygen consumption is simply the sum of membrane lung and native lung oxygen delivery:
=
+
.





Besides the oxygenation capability of both the membrane lung and patient’s lung, the main determinants of blood O 2 content are hemoglobin saturation and hemoglobin concentration. Once the hemoglobin has been saturated, the only way to increase O 2 delivery and O 2 transport is by increasing the hemoglobin concentration ( Fig. 103-4 , left panel).



Under VV-ECMO, arterial oxygenation will depend on the sum of the partial effects of , mixed venous S o 2 , and
.
O 2 Delivery by the Membrane Lung (
)
The amount of oxygen delivered by the membrane lung depends on three main factors (see Fig. 103-4 ).
- 1.
The intrinsic performance of the membrane lung. The oxygenator’s oxygenation capability will depend on the membrane diffusion characteristics (thickness, material) and the membrane surface area (see Fig. 103-4 , left panel).
- 2.
The O 2 pressure gradient between the sweep gas flow and the blood. On the gas side, the O 2 partial pressure depends on the F io 2 of the sweep gas. It is important to understand that, similarly to natural lung physiology, the transfer of O 2 through the membrane lung is governed by the ventilation/perfusion matching. However, once a high level of partial pressure has been reached in the outlet blood, further increase in gas flow will not lead to any major increase in O 2 delivery. On the blood side, the higher the oxygen saturation of incoming blood (S o 2in ), the lower the quantity of O 2 that can be added to the blood (see Fig. 103-4 , right panel). As described earlier, an important factor that may result in a high S o 2in is recirculation (i.e., blood already oxygenated from the membrane lung sucked back into the ECMO circuit).
- 3.
ECMO blood flow. Up to a determined blood flow,
will increase directly with the increase in ECMO flow. However, depending on the membrane surface area and the membrane lung characteristics, there is a limit to the oxygenator flow (“rated flow”) above which no more O 2 can be added to the blood (see Fig. 103-4 , left panel).
Mixed Venous Blood Oxygenation (
)
During VV-ECMO, the main determinants of mixed venous oxygen saturation ( ) are the oxygen saturation of the blood leaving the organs and the ratio between extracorporeal BF and CO ( Fig. 103-5 ). At a given CO, the increased oxygenation contribution of the membrane lung resulting from an increase in BF will always lead to an increase in
and arterial oxygenation saturation (Sa o 2 ). In contrast, when CO changes at constant BF, the effect on
and Sa o 2 is primarily dependent on the level of BF, Cvc o 2 , and recirculation. A decrease in CO is associated with a parallel decrease in Cvc o 2 : Below a certain value of Cvc o 2 , the expected positive effect on oxygenation of the increase in the BF/CO ratio may be offset by the decrease in Cvc o 2 , resulting in a net decrease of
and Sa o 2 . At BF commonly used in clinical practice (3 to 5 L/min), an increase in CO is commonly associated with a decrease in the oxygenation contribution of the membrane lung, resulting in a decrease in
(see the iso BF line with 4 L/min in Fig. 103-5 ) and ultimately a decrease in Sa o 2 ( Fig. 103-6C ). Conversely, at lower BF (1 to 3 L/min), and at critically low arterial oxygenation, an increase in CO is associated with an increase in both
(see the iso BF line with 1 L/min in Fig. 103-5 ) and Sa o 2 (see Fig. 103-6C ). These relationships are the basis for the manipulation of BF and CO during VV-ECMO. However, there are limitations to how much the BF/CO ratio can be increased. First, in the presence of recirculation, either an increase in BF or a decrease in CO will increase the amount of recirculating blood, thereby blunting the expected positive effect on oxygenation. Second, an increase of BF is limited by the cannula size and blood volume status of the patient. Third, oxygen delivery to peripheral organs depends on both Ca o 2 and CO; at low oxygenation levels, a higher CO is necessary to ensure sufficient organ oxygenation.





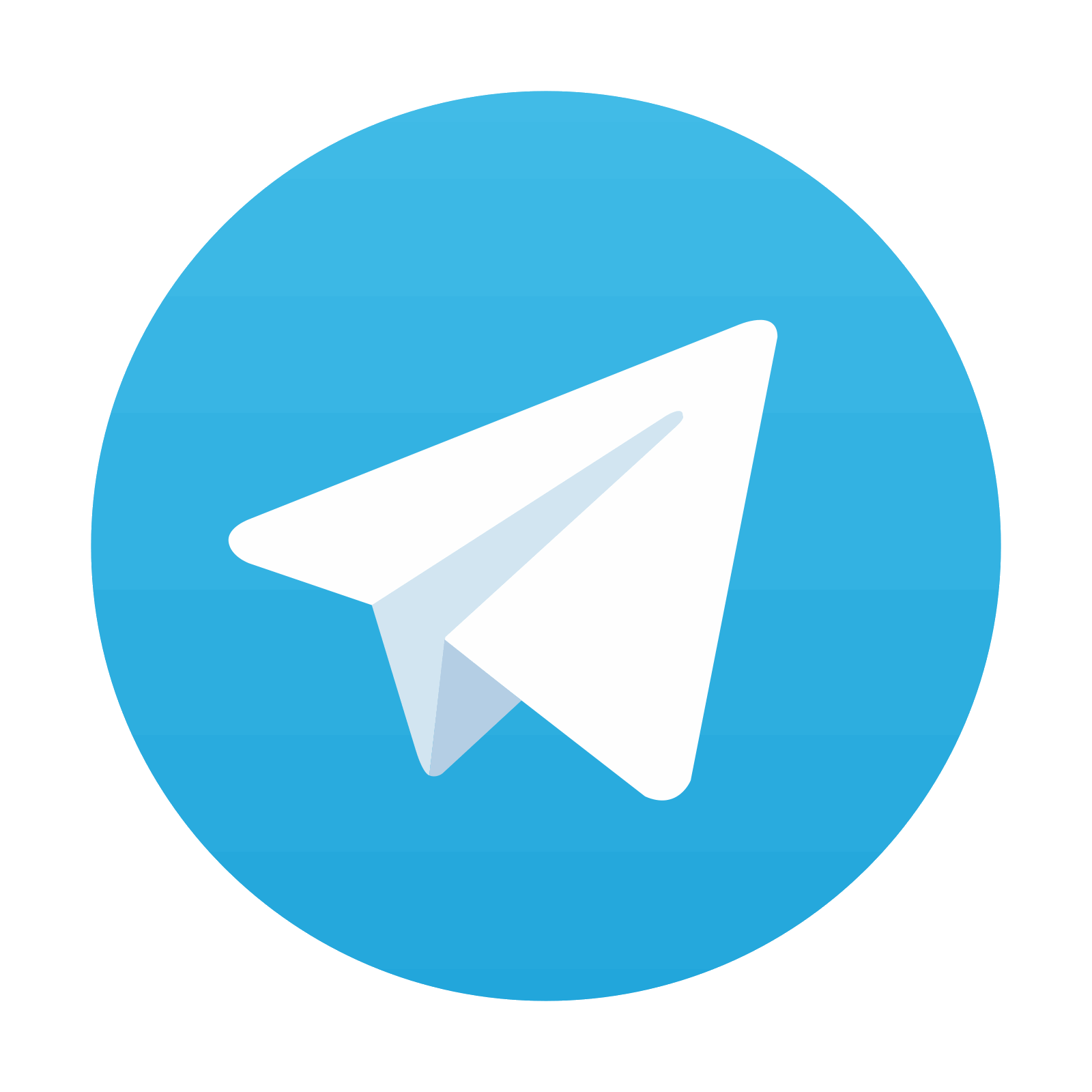
Stay updated, free articles. Join our Telegram channel
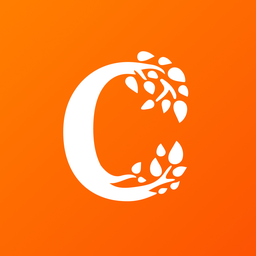
Full access? Get Clinical Tree
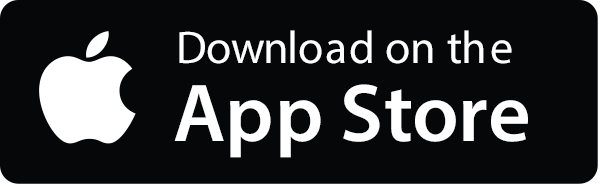
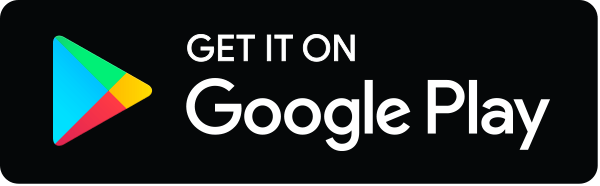
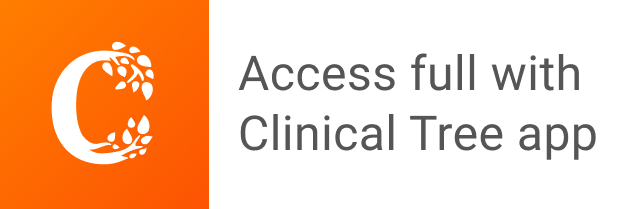