Evolving Early Detection Modalities in Lung Cancer Screening
Simon D. Spivack
William Rom
It would seem intuitively obvious that early detection efforts of a lethal disorder such as lung cancer would increase the chances for cure of that disease for individuals, and therefore, the population from which they derive, and thereby lead to a reduced death rate (reduced mortality) from that disease. This has proven to be the case for cervical and breast cancer screening.1,2 However, to date, no molecular nor imaging modality of the lung, although suggestive, has yet been sufficiently evaluated to incontrovertibly confirm that it reduces mortality for early lung cancer detection.3 Several studies of various lung imaging and other modalities are currently under way, are promising, and are described elsewhere in this textbook (see Chapters 15,16 and 17).
The lung is a visceral organ; therefore, access is necessarily limited. It is virtually impossible to directly examine all ramifications of the complex branching structures of the bronchial tree by external imaging, endobronchial fiberoptics, or other modalities. Therefore, certain aspects of a transforming epithelium must go unexamined, even by the most sophisticated sampling techniques. This inherent problem is reflected in imperfect sensitivity for all molecular lung cancer-screening modalities tested to date. Clearly, lung epithelial sampling approaches are evolving.
Also underappreciated is that any given screening modality will necessarily be imperfectly specific, in isolation, but two or more complementary stages in screening may, when coupled, offer vast improvements in predictive value of a positive test. That is, serial molecular monitoring for risk stratification could complement or even leverage imaging approaches to disease detection, by allowing enrichment of the disease prevalence (“risk”) of the population destined for disease detection by more specific imaging approaches. Thus, in a typical population of otherwise unselected middle-aged smokers and ex-smokers, where lung cancer prevalence is about 2%, the positive predictive value (PPV) of a noncalcified nodule detected on spiral computed tomography (CT)—where typically specificities are ∽90%—proves to be lung cancer in only about 18%.4 However, if one uses a biomarker of risk to identify a higher-risk portion of the population, where prevalence for lung cancer is 20%, the PPV (probability that same CT-detected nodule represents lung cancer in this context), is much greater at ∽70%.
Therefore, a staged approach to screening, first using a risk assessment tool, followed by a disease detection tool, implies that two different tiers or levels of performance can, when coupled, have synergistic effects on early detection efficacy. Here, the first tier of screening would identify less deterministic “risk factors,” perhaps both demographic and molecular. Such nondeterministic but informative risk-assessment tools abound in the literature but are often underappreciated as such. Then, the second tier testing would entail much more stringent, and specific, conventional performance criteria for actual lung cancer detection markers or imaging features.4
Candidate noninvasive risk assessment tools for lung cancer screening might simply collect readily available clinical information in sophisticated risk prediction models.5,6 Alternatively, as will be demonstrated, one can biologically sample the entire, or portions of, lung epithelium and include, for example, germline genetic polymorphisms in carcinogen metabolism or DNA repair genes (blood-or buccal cell-based); blood-based proteomic signatures; transcriptional signatures in a related airway specimen (e.g., brush-exfoliated buccal cells), sputum oncogene mutation, or tumor suppressor gene silencing (spontaneously exfoliating lung epithelial and inflammatory cells); or exhaled breath tests of volatile (alkanes or aldehydes) or nonvolatile compounds (DNA), derived from as yet undetermined airway origin (e.g., bronchial or alveolar lining fluids).
Conventional clinical disease detection tools might include anatomic-based CT scans, functional imaging such as positron emission tomography (PET) scans (see Chapter 27), various conventional and enhanced optical bronchoscopic techniques (see Chapters 19 and 28), or other localizing modalities, all discussed elsewhere in this text, followed by definitive tissue sampling where indicated, with attendant conventional or newer molecular assays applied. In this chapter, we will largely emphasize less invasive, molecular-oriented approaches to both
risk assessment and disease detection that are inherently more easily applied to an asymptomatic population determined by clinical history to have some propensity to lung cancer.
risk assessment and disease detection that are inherently more easily applied to an asymptomatic population determined by clinical history to have some propensity to lung cancer.
AIRWAY IMAGING APPROACHES
Bronchoscopic Optical Techniques Although invasive, fiberoptic bronchoscopy has been considered in certain high-risk lung cancer screening contexts. Autofluorescence bronchoscopy (AFB), based on altered refractile properties of multilayered hyperplastic or dysplastic epithelium, has proven superior to conventional white-light bronchoscopy (WLB) in detecting moderate-to-severe dysplasia, and carcinoma in situ with 56% to 82% sensitivity (AFB), versus 9% to 58% sensitivity (WLB).7,8 AFB is not universally available. Because there are several localized responses to detection of premalignancy and overt but confined bronchial malignancies (Nd:YAG laser therapy, photodynamic therapy [PDT], electrocautery, cryotherapy, and brachytherapy), some have advocated routine bronchoscopic screening of individuals determined as high risk by other means (e.g., high-grade sputum atypia, chronic obstructive pulmonary disease [COPD], asbestos exposure).9,10 Specificity of AFB has been a question. Advancement of WLB techniques, including light-scattering technologies and videobronchoscopy, is imminent.4,11,12,13,14 High-density radiographic CT image reconstruction has allowed for the development of virtual bronchoscopy, a technique that is completely noninvasive, although radiation dose is not trivial, and radiologist/computer evaluation strategies still evolving15 (see Chapters 19 and 28).
LUNG TISSUE-BASED MOLECULAR ASSAYS
Of course, the gold standard for lung cancer detection is examination of lung tissue itself, either by conventional means (microscopy, immunohistochemistry, and the like), or by newer molecular means. Among the newer means, fluorescence in situ hybridization (FISH) for larger-region genomic deletions or duplications (see Chapter 49), microsatellite examinations (see Chapter 7), DNA methylation patterns (see Chapter 7), microarray messenger RNA (mRNA) expression signatures, candidate mRNA expression signatures, microRNA microarrays, candidate microRNA expression signatures, proteomic and antibody arrays, and metabolomic approaches are all in use in experimental and/or beta-testing for early clinical application. The National Cancer Institute (NCI) empowered, in the last decade, the Early Detection Research Network (EDRN) to create a consortium of tissue repositories and technology platforms for this purpose, and the technologies presented at the most recent 2008 meeting are truly astonishing. As this chapter emphasizes evolving early detection modalities, largely by molecular assays on noninvasively obtained tissues from individuals without symptoms, those molecular techniques requiring robust, large surgical level tissue specimens are covered elsewhere in this volume.
For noninvasively obtained tissues used in early detection studies on asymptomatic populations, such as exfoliated cells in sputum, or the circulating macromolecules of blood, polymerase chain reaction (PCR)-based assays of DNA and RNA are most often employed in the translational research setting. Their sensitivity is extraordinary, allowing analysis of these lung-surrogate tissues with greater ease. It must be acknowledged that although conferring great sensitivity, these nucleic acid end points may, even for cancer syndromes, often be considered more biologically proximate, or twice-removed end points, as opposed to direct measurement of the full-dimensional cancer biological phenotype (uncontrolled growth, invasion, metastasis), which is generally not feasible. Even other proximate markers (metabolites or proteins/enzymes generating those metabolites) that are the direct underpinnings of these cancer phenotypes are often beyond the level of sensitivity of current assays, although proteomic, and antibody microarray technologies are advancing rapidly as are informatic approaches to these multidimensional data sets.
DNA-based genome-wide search for aberrant copy number in tumors have revealed copy number aberrations using single nucleotide polymorphism (SNP) arrays or array comparative genomic hybridization (CGH) and reveal that many of the copy number alterations found to cluster in lung cancer involve unknown genes.16
DNA-based methylation markers in lung tissue, by example: cytosine-phosphodiester-guanine (CpG) methylation of gene promoter DNA is a major correlate of biologic pathway regulation and deregulation.17,18,19 In many ways, progressive DNA methylation of tumor suppressor genes has been revealed over the last 10 to 15 years to be a strong correlate of lung carcinogenesis.
As a recent example of a comprehensive methylation biomarker search, a concerted genome-wide effort to assay in vitro genes likely to be methylated, and responsive to a methylation inhibitor, was checked for consistency with expression and methylation profiles in human lung. Those genes whose expression was most discriminatory for lung tumor from adjacent nontumor tissue (for lung, specifically) by methylation-specific PCR (MSP) sampling of one or a few (CpG) methylation sites in each gene locus, included BNC1, MSX1, CCNA1, RASSF1A, p16, ALDH1A13, LOX, and CTSZ. This is one of the first such comprehensive genome-wide searches for methylation-silenced genes in lung cancer20 and has been followed up by others.21
In another study, a panel of candidate gene DNA methylation markers, using methylation-specific PCR sampling was constructed, and using the top four markers (CDKN2A EX2, CDX2, HOXA1, OPCML) could distinguish lung tumors from normal tissue with 94% sensitivity and 90% specificity.22
A workshop was convened in 2005 on the use of methylated DNA sequences as cancer biomarkers, in risk assessment and disease detection, enjoining the NCI’s EDRN and the National Institute of Standards and Technology. The summary23 has emphasized the importance of specimen choice and handling, bisulfite modification strategy, choice of assay based on scale, sensitivity and cost, tissue specificity, and other factors. These issues remain at play for current lung cancer screening biomarkers (see Chapter 7).
BLOOD-BASED MARKERS
Blood is an attractive surrogate specimen source because of its availability and the presumed pooling of metabolic processes in the circulation, as reflected, it is postulated, in that compartment.
DNA-Based Markers in Blood The quantity of DNA leaching into the blood itself has been proposed as a marker,24 albeit controversially.25,26 Attempts to detect DNA-based somatic markers in the blood have been myriad,27 including oncogene mutations, somatic mutations, and other modifications, with mixed results. For the most proximate steps in tobacco carcinogenesis, the measurement of DNA adducts of known tobacco carcinogens in blood cells, several large European studies using 32P postlabeling of adducts did not show consistent relation to smoking, nor risk.28,29 A more recent large, pooled data analysis also suggests a weak association of bulky DNA adducts and lung cancer, perhaps apparent in current and recent smokers only.30
Aberrant DNA methylation in blood has been detected for the last decade, albeit its origin remains unclear, but these have been as disappointing as diagnostic tools.31,32,33
For risk assessment, blood-based genome-wide association and family linkage studies for germline variants associated with lung cancer have emerged, although in somewhat sparse number. A susceptibility locus on chromosome 6q23-25 was reported from a multi-institutional consortium examining high-risk family pedigrees, led by National Institute of Health (NIH) investigators34 (see Chapter 4). The individual candidate genes in the region have not yet been confirmed with regard to association of their variants with lung cancer risk.
Individual variants in component enzymes in specific pathways have been explored in some detail in cross-sectional, case-control population-based studies, with particular attention paid to carcinogen metabolism,35 DNA repair,36,37,38 and inflammation.39 In general, these data for individual variants have been inconsistent across populations but may have some incremental information when integrated into comprehensive risk assessment models that include demographic data. These are reviewed in greater detail in the cited references.
RNA-Based Markers in Blood There have been a small number of well-executed and suggestive studies of RNA expression in recovered circulating tumor cells40,41,42 and/or peripheral blood lymphocytes.43 Free circulating RNA studies are inherently challenging for quality template recovery, RNA-specific amplification, and detection of signal above background.
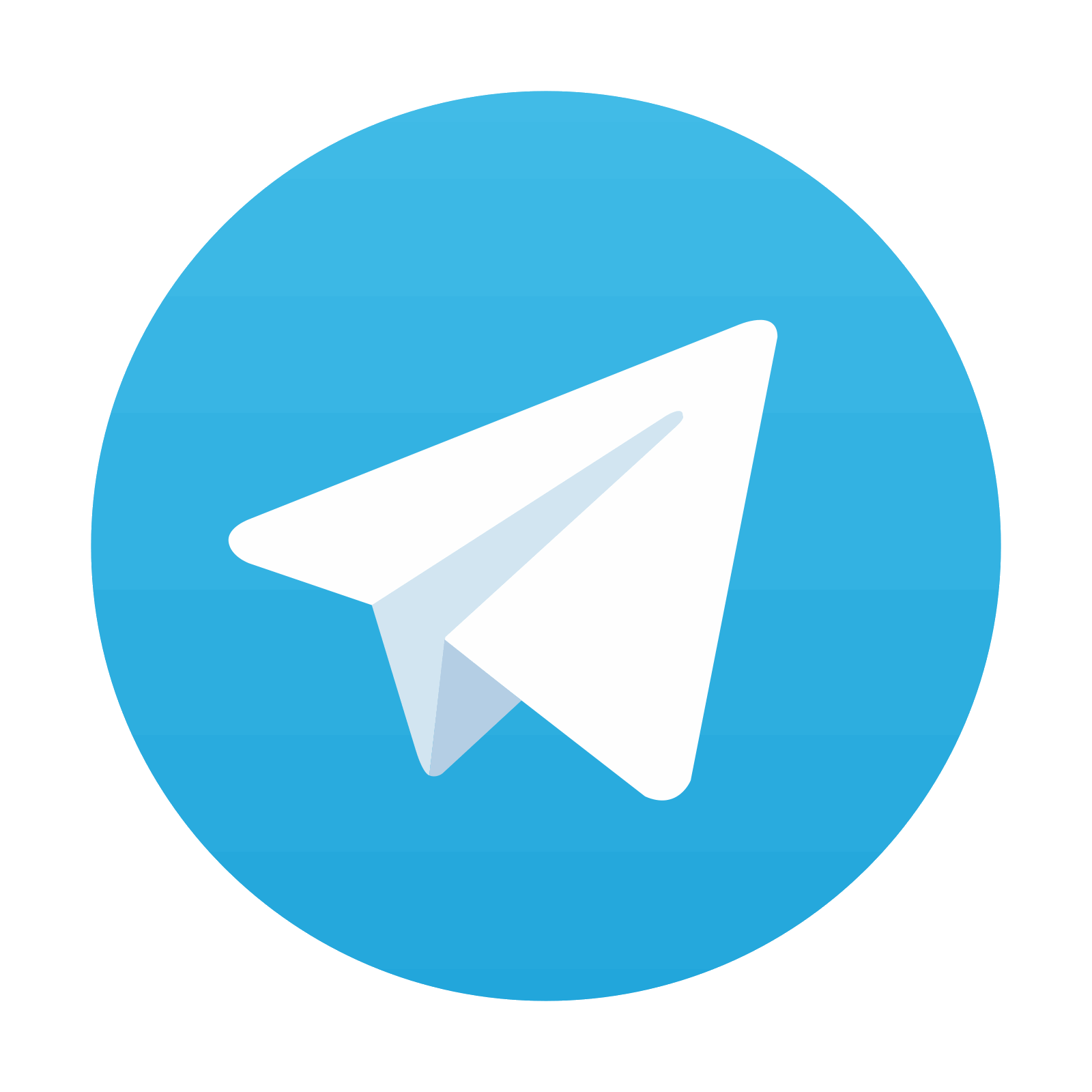
Stay updated, free articles. Join our Telegram channel
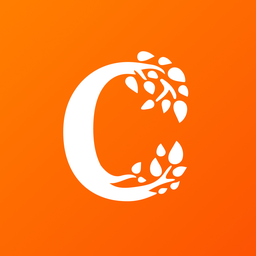
Full access? Get Clinical Tree
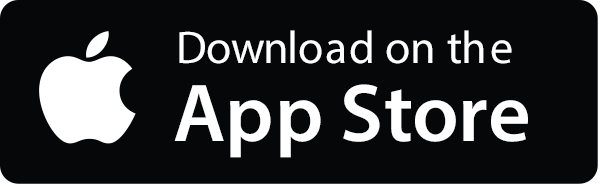
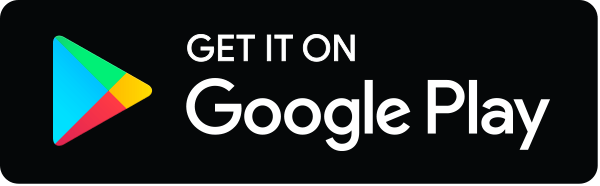