(A) α4 nAChR immunostaining (purple) in airway epithelial cells and submucosal glands (200x); (B) β2 immunostaining (purple) in airway epithelial cells and submucosal glands (200x) (C) α7 (red) immunostaining in airway epithelial cells and airway fibroblasts (100x). (D) Choline acetyltransferase (ChAT) immunostaining in red, PGP a nerve fiber marker in green. ChAT, the enzyme that synthesizes ACh is clearly present in airway epithelial cells and distinct from nerve fibers. aw = airway, smg = submucosal gland.
Treatment of pregnant rhesus monkeys with low levels of nicotine designed to simulate the nicotine exposure of pregnant human smokers increased levels of α7 nAChR in airway epithelial cells and fibroblasts in fetal monkey lung (Figure 5-2) and increased collagen in a similar distribution (25,30,31). As observed in studies in rodents, prenatal nicotine exposure decreased levels of elastin (Figure 5-2). In the rat model, nicotine caused lung hypoplasia, reduced surface complexity of developing alveoli, caused discordant growth of the airways and alveoli, and increased the number of alveolar type II epithelial cells. Consistent with increased type II cells, the expression of surfactant protein B (SP-B) and SP-A were increased (25).
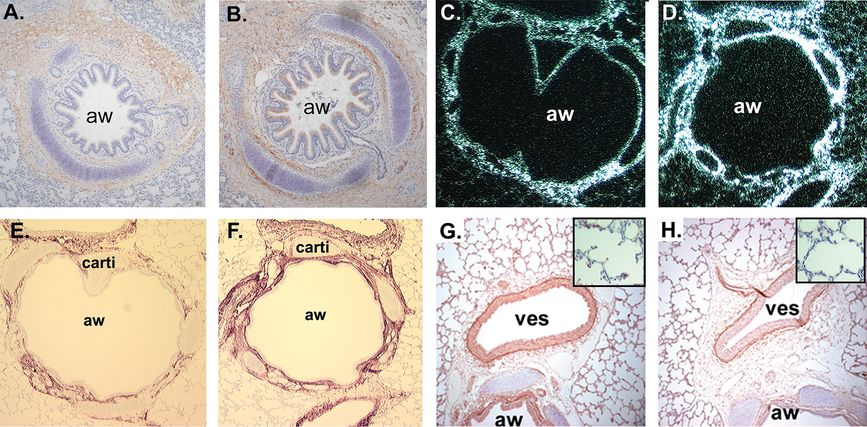
(A) α7 nAChR immunostaining of 134-day fetal lung. Chromogen = AEC (red), 100X. (B) α7 nAChR immunostaining of 134-day nicotine-exposed lung. (C, D) In situ hybridization showing collagen α1(III) mRNA expression in lung from control and nicotine-exposed animals (100X). (E, F) Collagen III immunostaining in lungs from control and nicotine-exposed animals. Chromogen = AEC (red), 100x. aw = airway, carti = cartilage. (G, H) Elastin immunostaining in airway associated vessels from control and nicotine-exposed animals. Insert boxes show elastin staining in tips of secondary septi (400x).
Nicotine-induced changes in lung structure translated into alterations in pulmonary function in the newborn rhesus monkeys similar to those measured in children born to smoking mothers (Figure 5-3) (32). In the pregnant rhesus model, dams were infused subcutaneously with nicotine at 1.5 mg/kg/day or saline from days 26 to 160 of gestation (term is 165 days). Cesarean sections were done at 160 days and pulmonary function measured at 24 hours of age. Nicotine significantly decreased lung volume and forced expiratory volume (FEV 0.2), peak tidal expiratory flow during tidal breathing, and mean mid-expiratory flow (MMEF or FEF 25%–75%) when compared to saline controls. Pulmonary resistance was significantly increased while static and dynamic lung compliance decreased, but not significantly (Figure 5-3) (32). Increased collagen and decreased elastin caused by prenatal nicotine exposure likely underlies the decreased compliance caused by nicotine (Figure 5-2).
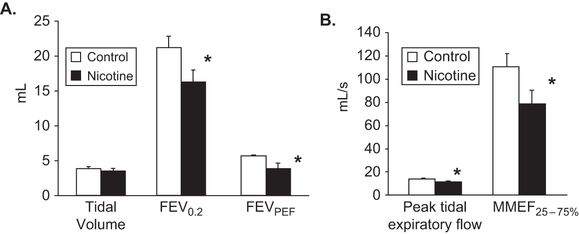
Values are means ± SE. FEV0.2 (forced expiratory volume during first 200 milliseconds, FEVPEF (forced expiratory volume at peak expiratory flow), MMEF25–75% (mean mid-expiratory flow). *p < .05 compared to control.
Changes in pulmonary function caused by prenatal nicotine exposure were similar to those seen in human infants exposed to maternal smoking during pregnancy. These findings strongly suggest that nicotine is the component in tobacco smoke that impairs normal lung development and leads to decreased pulmonary function in offspring of women who smoked during pregnancy.
Studies in mice provide a potential mechanism by which prenatal nicotine exposure leads to decreased expiratory flow. In embryonic murine lung explants cultured in vitro, nicotine stimulated lung branching and caused dysanaptic lung growth in a dose-dependent fashion. Effects of nicotine were dependent on the presence of the α7 nAChR receptors (33). In a murine model of in utero nicotine exposure in which pregnant mice were treated with nicotine from gestation day 7 to postnatal day 14, pre- and postnatal nicotine exposure significantly decreased forced expiratory flows in the offspring, findings similar to those in humans and monkeys (34). Effects of nicotine were blocked in α7 nAChR gene deleted mice (34). When mice were exposed to nicotine during gestation days 7–21, gestation days 14 to postnatal day 7, and postnatal days 3–15, only exposure from prenatal day 14 to postnatal day 7 decreased forced expiratory flows in the offspring (Figure 5-4) (34). This time period in mouse lung development includes the pseudoglandular, canalicular, and saccular periods, but occurs before most of the alveolar period (35), a finding supporting a primary effect of nicotine on airway growth. Stereologic analysis of airway size and diameter showed an increased number of airways of small diameter after nicotine exposure (Figure 5-5). These data support the concept that prenatal nicotine exposure decreased forced expiratory flows by simulating epithelial cell growth and altering airway morphogenesis to resulting in longer and more torturous airways; thus forcing airflows through narrower tubes. After prenatal nicotine, exposure to methacholine caused increased airflow resistance in adulthood, even in the absence of allergic sensitization, consistent with a link between maternal smoking during pregnancy and increased risk of childhood asthma.
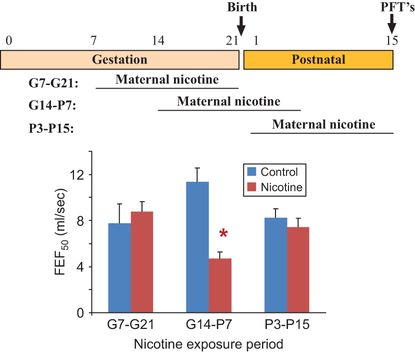
C57BL6/J mice were mated and pregnant/lactating females treated with nicotine (2 mg/kg/day) for 2-week windows of exposure from gestation day 7 to gestation day 21, gestation day 14 to postnatal day 7 and from postnatal day 3 to postnatal day 15. On postnatal day 15, mice were evaluated by pulmonary function testing as previously described (34). Only wild-type mice treated with nicotine from gestation day 14 to postnatal day 7 had significant decreases in forced expiratory flows. Error bars denote ± SD,*p < 0.05 compared to control, n = 6–8 animals in each group. (FEF50 = forced expiratory flows at 50 msec.) Similar effects were also seen for FEF75.
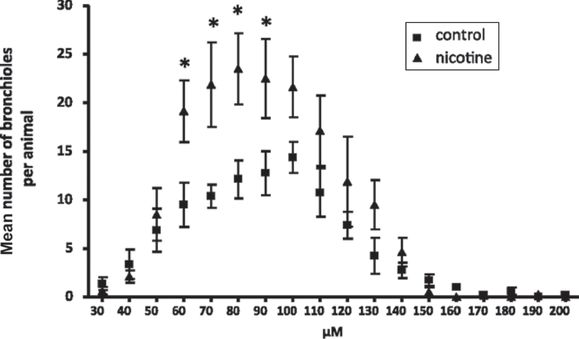
C57BL6/J female mice were administered either water or water containing 100 μg/mL nicotine before timed breeding and throughout gestation. Lungs were from age-matched offspring at 8 weeks of age. Whole lungs were embedded and sectioned in an isotropic, uniform random manner to create unbiased samples for examination. Airway diameter was measured for each visible bronchus and bronchiole. Mice exposed to prenatal nicotine had significantly greater numbers of small bronchioles, with diameters between 60 and 90 μm (n = 8 per group). Error bars denote SD. *P < 0.05 compared with control.
Thus, based on data from animal models, the effects of smoking during pregnancy on pulmonary function in the offspring are likely mediated by nicotine acting to stimulate nicotinic receptors expressed in the developing lung, mediated in part by α7-nAChR. Effects of nicotine are associated with increased collagen production and altered airway geometry at critical periods in the latter part of pregnancy.
Clinical Sequela of In Utero Tobacco Exposure
Multiple studies have shown increased lower respiratory illness in infants born to mothers who smoke (36–39). Taylor and Wadsworth (38) studied 12,743 children and found significantly increased bronchitis and hospital admissions for lower respiratory illness in children from smoking mothers. Tager et al. (40) found increased lower respiratory illness with prenatal smoke exposure but not with postnatal exposure. Increased wheezing and asthma in children born to mothers who smoked during pregnancy have been repeatedly documented (11,41–46). Prenatal exposure is more deleterious than postnatal exposure. A large meta-analysis drawing data from 79 prospective studies (47) estimated the effects of prenatal and postnatal smoking by the mother, the father, or any other household member during three different age ranges of the offspring: 0–2, 3–4, and 5–18 years of age. Prenatal maternal smoking had the strongest effect on asthma in children ≤ 2 years old. A pooled analysis of eight European birth cohorts (48) demonstrated that maternal smoking during pregnancy increased the risk of wheeze and asthma among children who were not exposed to maternal smoking after birth. A recent study also showed a highly significant interaction between LBW, maternal smoking during pregnancy, and risk of the offspring developing asthma (45).
There appears to be a direct link between decreased indices of offspring pulmonary function (primarily decreases in forced expiratory flows in the small airways) caused by maternal smoking during pregnancy and increased rates of respiratory illness. Decreased pulmonary function in infants was correlated with increased rates of respiratory illness (40,49–55). A prospective birth cohort study of 802 healthy babies in Norway (52), with follow-up from the newborn period through 10 years of age, demonstrated that infants with measurements of TPTEF: TE at or below the median shortly after birth have a history of asthma (24.3% vs. 16.2%, p = 0.01; OR of 1.58); have current asthma (14.6% vs. 7.5%, p = 0.005; OR of 2.10); and have severe bronchial hyperresponsiveness (9.1% vs. 4.9%, p = 0.05) at 10 years of age (52). From this same cohort, infants whose passive respiratory compliance was at or below the median shortly after birth were more likely to have a history of asthma (27.4% vs. 14.8%, p = 0.001; OR of 2.18) and current asthma (15.0% vs. 7.7%, p = 0.009; OR of 2.01). Similarly, Tager et al. (40) showed that the decreased VmaxFRC seen with prenatal smoke exposure correlated directly with increased lower respiratory illnesses (LRIs). An increased risk of wheezing in the first years of life was reported in children with a decreased ratio of TPTEF: TE measured in the first week of life (53) or at 3 months of age (50,53), and in those with reduced VmaxFRC at 1, 3, and 6 months of age (40,50,54,56). Another population-based longitudinal study demonstrated that infants less than 6 months of age with a decreased VmaxFRC developed wheezing and lower respiratory tract illnesses in the first year of life (40). In addition, results from the Tucson Children’s Respiratory Study demonstrated that children in the lowest quartile of pulmonary function continue to have decreased pulmonary functions as young adults, putting them at increased risk for developing chronic obstructive pulmonary disease (COPD) as adults (55,57).
These findings emphasize the importance of in utero or early life interventions to promote optimal lung development and function. A recent double-blind study (58) randomized pregnant smokers to daily vitamin C (500 mg/day) versus placebo during pregnancy and demonstrated that offspring born to women who received vitamin C had significantly improved newborn pulmonary function tests and decreased wheezing through 1 year of age. These findings suggest that in utero interventions offer the potential to influence factors related to the fetal origins of childhood and adult respiratory disease.
Implications for Nicotine Replacement Therapy During Pregnancy
A variety of nicotine replacement products, including nicotine-containing gums, patches, lozenges, and sprays, are currently available to potentially reduce the craving for smoking and thereby potentially decrease the pregnant smoker’s exposure to other toxins present in cigarette smoke. Unfortunately, there is a strong body of evidence that nicotine itself is an important mediator of the adverse effects on the fetal lung. There is no evidence supporting a safe dose for nicotine replacement therapy during pregnancy. In addition, there is insufficient evidence from randomized trials of nicotine replacement therapy (NRT) to determine whether or not NRT is effective or safe to promote smoking cessation during pregnancy and whether it has positive or negative impacts on birth outcomes. Coleman et al. randomized 1,050 pregnant smokers to active nicotine patches versus placebo patches. Both groups also received behavioral cessation support. There was no difference in the rate of abstinence from the quit date until delivery between the two groups, although the compliance of both groups was low. A recent Cochrane review (59) examined the safety and efficacy of six trials of NRT in 1,745 pregnant smokers. No significant differences for smoking cessation or other important birth-related outcomes were demonstrated between randomized groups.
Secondhand Tobacco Smoke Exposure
Despite the implementation of laws requiring smoke-free public and working places, secondhand tobacco exposure remains a significant burden to those who do not actively smoke. This is particularly true for developing fetuses and young children who spend the majority of time in their home environment, where secondhand smoke (SHS) exposure is present. Multinational studies report that up to 40% of pregnant nonsmokers are exposed to SHS due to smokers in their home environment. SHS consists of exhaled smoke as well as sidestream smoke that is released from a burning cigarette between inhalations, both of which have very similar compositions. Although the composition of SHS changes as it is diluted with ambient air in the environment and interacts with other compounds, SHS continues to contain significant amounts of nicotine (60). The effects of SHS exposure during pregnancy on lung development will likely depend on the relative amounts of nicotine and other toxins crossing the placenta. There is evidence from a recent meta-analysis that exposure of pregnant nonsmokers to SHS can reduce the mean birth weight of offspring by up to 33 grams and increase the risk of birth weight by less than 2,500 grams (61).
Because most pregnant nonsmokers who are exposed prenatally to a smoking home will likely continue this exposure postnatally, it is difficult to identify the specific effects of prenatal and postnatal SHS exposure on fetal lung development and postnatal respiratory health. A population-based cohort of 5,619 7-year-old Toronto children was evaluated for the longitudinal association between maternal smoke exposure in pregnancy (active smoker and passive exposure) and childhood asthma development by parental report (62). The children whose mothers smoked or were exposed to home secondhand smoke during pregnancy were more likely to develop asthma with an adjusted hazard ratio of 1.3. This association persisted for children of nonsmoking mothers exposed to SHS in the home during pregnancy after adjusting for SHS exposure from birth to age 7 years.
The impact of perinatal SHS exposure on airway obstruction and hyperresponsiveness has been investigated in a rat model (63) in which pregnant Sprague-Drawly rats were exposed to filtered air or to sidestream smoke in utero and/or postnatally for 4 hours per day, 7 days per week from day 3 of gestation until birth. The pups were exposed to filtered air containing sidestream smoke for 7–10 weeks postnatally. Rats exposed both prenatally and postnatally to sidestream smoke had significantly lower dynamic compliance and significantly greater reactivity to methacholine than those exposed to the other three treatments. Both pre- and postnatal exposure to sidestream smoke was needed to change pulmonary function (63). The effect of perinatal SHS was studied by Joad et al. at the California National Primate Center in a nonhuman primate model, in which pregnant rhesus monkeys were exposed to filtered air or SHS for 6 hours per day. Exposure for 5 days per week started at 50 days of gestational age and continued postnatally until 3 months of age altered with intrinsic airway responsiveness and alveolar attachments (64).
E-cigarettes and Pregnancy
Electronic cigarettes (E-cigarettes) are tobacco-free nicotine delivery devices in which burning tobacco is replaced by a battery-operated atomizer that produces an aerosol from a liquid containing nicotine and flavoring. The liquid used to solubilize nicotine contains carrier solvents such as glycerol and/or propylene glycol, which deliver the nicotine and flavorings directly into the respiratory tract. Potential effects of e-cigarettes on lung development will include exposure to nicotine and production of toxins from the carriers. E-cigarette use during pregnancy exposes the mother and fetus to nicotine and potential toxicants in an aerosol and includes the potential risks associated with secondhand exposure to e-cigarette vapor. Aerosols from e-cigarettes contain toxic and carcinogenic carbonyl compounds such as formaldehyde, acetaldehyde, and acrolein (65–68). In particular, levels of formaldehyde were comparable to those measured in regular cigarette smoke. Levels of these compounds vary dramatically, depending on the brand or model of e-cigarette and the formulation of the aerosol liquids. E-cigarette vapor and secondhand e-cigarette vapor may affect lung development in a manner similar to smoking and are therefore likely risks to the infants of mothers using e-cigarettes.
A major concern with e-cigarettes is their potential to deliver nicotine during pregnancy. Progression toward nicotine dependence associated with e-cigarettes is still unclear. Although there appears to be a learning curve for effective “vaping” (69,70), experienced users of e-cigarettes achieve levels of nicotine and cotinine similar to individuals using conventional cigarettes (69–71). E-cigarettes have the potential to deliver as much nicotine as conventional cigarettes and therefore to alter lung development in similar fashion. It is likely that e-cigarettes will prove to be as addictive as conventional cigarettes. Advertising conveys the concept that e-cigarette usage is safe, but their use may lead to addiction in vulnerable individuals who will continue use of e-cigarettes during pregnancy. Use of e-cigarettes in adolescents is a particular concern because they may be at greater risk to develop nicotine addiction and to continue the use of e-cigarettes during pregnancy (72–75).
Environmental Pollution
Increased environmental air pollution is associated with increased risk of childhood asthma, respiratory infections, and indices of reduced lung function(76–79). Various types of air pollution, including particulate matter, ozone, nitrogen oxides (NO), sulfur oxides, and carbon monoxide (CO) have been associated with altered lung development. Environmental air pollution is associated with increased risk of premature delivery and intrauterine growth restriction (80–82). Effects on birth weight have been summarized by Proietti et al. (83). For most of the epidemiologic studies on the effects of air pollution on lung development, it is difficult to differentiate prenatal from early childhood effects.
In a study by Jedrychowski et al. (84), children in Poland exposed to the highest quartile of PM2.5 (inhalable material <2.5 μm in diameter) had significant decreases in FVC, FEV1, and FEV0.5. The increased exposure to PM2.5 was in turn associated with increased wheezing at 2 years of age, though this effect was no longer significant by 4 years of age (85). Mortimer et al. (86) identified negative associations among pulmonary function with prenatal and early exposures to PM10, NO, and CO in asthmatic children, although associations were for specific subgroups of children (86). Exposure to increased levels of CO during pregnancy increased allergic sensitization in children with asthma (87). In a retrospective study of 37,401 children born in British Columbia by Clark et al. (88), the incidence of asthma in 3- to 4-year-olds was correlated with estimated levels of in utero and first year of life exposures to air pollution. There was an increase in asthma risk with increased exposure to NO, CO, and PM10.
The mechanisms by which exposure to air pollution alter lung development and lead to increased respiratory disease are not completely clear, although animal models have been developed to examine effects of both particulate and gaseous pollution. Fedulov et al. (77) showed that exposure of pregnant mice to diesel exhaust particles, as well as to inert particles, resulted in increased airway reactivity in the offspring. Plopper, Schelegle, and colleagues at the California National Primate Research Center developed models of ozone exposure to infant monkeys to determine how ozone affects lung development and leads to lung disease. Ozone is a strong oxidant and is a major component of air pollution in cites. Ozone is formed by the interaction of sunlight with hydrocarbons and nitrogen oxides produced during combustion. Exposure of infant monkeys to ozone caused decreased lung branching, hyperplastic airway epithelium, alterations in alveolar development, and smooth muscle remodeling (89). The combination of ozone and allergen increased the innervation and increased CD25+ cells of the airway epithelium (90,91), providing a potential link between ozone and asthma. Miller and coworkers have suggested that early exposure to ozone causes long lasting changes in innate immunity that may be regulated by changes in miRNAs, potentially miR-149 (92). Auten et al. (93) reported that prenatal exposure to diesel exhaust particles further enhanced airway reactivity and abnormalities in alveolar development. Thus, there is likely considerable interaction between multiple pollutants among pre- and postnatal exposures.
Indoor Air Pollution: Biomass Fuel Exposure, Wood Smoke, and Cookstoves
Over half of the world’s population (an estimated 3 billion people) and 90% of households in rural areas of developing countries burn unprocessed biomass fuel, typically wood, charcoal, dried animal dung, and agricultural residues. Incomplete combustion results in gaseous air pollutants and fine particulate matter (PM) that linger in the cooking area. Women and children in low- and middle-income countries are disproportionately impacted by exposure to high levels of indoor air pollution related to their role in cooking for the family. Significant increases in respiratory diseases including doubling of the risk of pneumonia and other acute lower respiratory infections in children <5 years of age (94) and an increased risk of COPD, asthma, and tuberculosis in adults have been reported (95). A recent meta-analysis (95) extracting data from 25 studies demonstrated an overall pooled OR that indicated a significant association of solid biomass fuels with acute respiratory infections in children (OR 3.53, 95% CI 1.94 to 6.43). No significant association with asthma was noted; however, only four appropriate studies in children were available for analysis (95). Although tobacco smoking is an established risk factor for COPD, 25–45% of patients with COPD have never smoked. Emerging evidence suggests that biomass exposure may be among the biggest risk factors for COPD globally (96).
The relationship between biomass use and adverse pregnancy outcomes/abnormal lung development is only partially understood. Because smoke from biomass cooking and heating produces many of the same pollutants found in tobacco smoke and outdoor air pollution, there is good reason to expect a relationship between exposure to biomass smoke and adverse lung development. Like tobacco, smoke from biomass combustion produces a large number of health-damaging air pollutants, including PM, carbon monoxide, nitrogen oxides, formaldehyde, benzene, 1,3 butadiene, polycyclic aromatic hydrocarbons, and many other toxic organic compounds (97). Carbon monoxide binds to hemoglobin-forming carboxyhemoglobin, which reduces the ability of blood to carry oxygen with a potential to cause intrauterine growth restriction (IUGR), reduced birth weight, or perinatal mortality. There is evidence for probable in utero effects of biomass exposure. Analysis of 3,559 childbirths in Zimbabwe demonstrated that babies born to mothers cooking with wood, dung, or straw were 175 grams lighter compared to babies born to mothers using liquefied petroleum gas, natural gas, or electricity (98). A population-based cohort of 11,728 live born infants were followed from birth through 6 months of age in India, and exposure to biomass fuel was associated with an adjusted 49% increased risk of LBW, a 34% increased incidence of respiratory illness, and a 12% increased risk of 6-month infant mortality (99). The mean BW was 104.5 grams lower in infants born to women from biomass using households (99). A study in India demonstrated a significant increase in stillbirths associated with cooking with biomass fuels (OR = 1.44; 95% CI: 1.04–1.97) (100). A significant increase in severe stunting (height at 2 standard deviations below the median of an international reference population recommended by the World Health Organization) and moderate to severe anemia (hemoglobin < 9.9 g/dL) was associated with biomass fuel use in India (101). IUGR has been associated with reduced lung function in infants (102), children (103), and adults (104), indicating it may influence lung function throughout life. Indeed, exposure to biomass smoke was associated with deficits in lung function (FEV1, FVC, FEV1/FVC, and forced expiratory flow at 25–75% of FVC), an effect that can be detected as early as the late teenage years, suggesting a detrimental effect of biomass smoke exposure on lung growth in early life (105).
PM in biomass smoke may increase the chance of an adverse pregnancy outcome by reducing the mother’s lung function and increasing maternal risk for chronic and acute respiratory diseases that may reduce oxygen delivery to the fetus. In biomass burning households, the PM10 and PM2.5 often exceed guideline levels of mean 24-hour concentration, especially during cooking (106). Histologic sections of adult lungs have shown that ambient PM penetrates into and is retained in the walls of small airways. Even in nonsmokers, long-term exposure to high levels of ambient particulate pollutants is associated with small airway remodeling that may produce chronic airflow obstruction (107). A controlled trial in households in Mexico randomized to Patsari stoves (that reduce indoor emissions) versus traditional open fires demonstrated that the Patsari stove was associated with significantly lower risk of respiratory symptoms, including cough and wheezing, and a lower FEV1 decline over a 1-year follow-up compared to open fire use in women over 20 years of age (108). This reduction of respiratory symptoms and of slower lung function decline was comparable to smoking cessation (108). Polycyclic aromatic hydrocarbons (PAHs) are also important potential toxins present in biomass combustion. Some of the individual PAHs are classified as Class II carcinogens, and benzopyrene is classified as a class I carcinogen. As a whole, PACs have been associated with immunotoxicity (109). Women who cook exclusively with wood or kerosene have higher creatinine-adjusted hydroxyl-PAH levels in their urine samples compared to women who cook with liquefied petroleum gas or coal briquettes (109). In a subset of the women in the previous trial who were randomized to the use of the Patsari stove versus open fire cooking, use of the Patsari stove also significantly reduced carbon monoxide and polycyclic aromatic hydrocarbon exposures (110).
Although the relationship between indoor air pollution and increased respiratory disease appears well established, our understanding of the underlying molecular and cellular events culminating in the pulmonary response is limited and evolving. Exposure to wood smoke PM in cell cultures is associated with increased expression and production of proinflammatory cytokines, oxidatively damaged DNA, and oxidative stress (111). An emerging hypothesis is that chronic PM exposure causes oxidative stress, reduced immunity, and subsequent infection. The oxidative potential of PM collected during the burning of wood and mixed biomass was evaluated with a validated, synthetic respiratory tract lining fluid. Incubation of this fluid with PM for 4 hours caused large losses of ascorbate and reduced glutathione, both physiologically relevant antioxidants (112). Short-term controlled exposure to high concentrations of wood smoke with intermittent exercise was associated with increased arterial stiffness and decreased heart rate variability in humans (113).
Studies in humans with regard to mechanisms have been less clear cut. A recent study in humans examining high inhalation exposure to wood combustion for a 1-week period demonstrated limited systemic effects in terms of inflammation, monocyte activation, and oxidative stress to DNA (114). Controlled woodstove exposure for 3-hour periods for 2-week intervals in atopic subjects did not alter markers of oxidative stress, DNA damage, cell adhesion, cytokines, or microvascular function (115). Also, short-term exposure to wood smoke at a concentration normally found in a residential area with a high density of burning woodstoves caused only mild inflammatory responses (116). Nevertheless, the role of environmental smoke will likely continue to be an area of active investigation.
Genetic Susceptibility
Both genetic polymorphisms and epigenetic factors regulating sensitivity of the lung to environmental exposures are likely strong factors that modify the effects of the environment or the outcome of programming and subsequent lung function in the offspring. Underlying the increased genetic susceptibility to environmental effects on lung development may be genetic polymorphisms that alter coding regions of genes either through deletions or single nucleotide polymorphisms (117). Most often these represent mutations in genes that either defend against the specific effector or genes that mediate the effects of the specific effector. Genetic polymorphisms can occur in either the mother, the fetus, or both. This concept is well illustrated by genetic polymorphisms that mediate the sensitivity of the fetus to maternal smoking during pregnancy.
Effects of maternal smoking during pregnancy are strongly mediated by nicotine. A polymorphism in the α5 nAChR, in which amino acid 398 is changed from Asp to Asn, increases the risk of smoking-related diseases such as lung cancer, COPD, and the degree of nicotine addiction (118). This same polymorphism in the mother increases the sensitivity of the fetus to the effects of maternal smoking during pregnancy on lung development. As shown in Figure 5–6, infants whose mothers smoked during pregnancy had diminished pulmonary function if their mothers were heterozygous or homozygous for the α5 risk allele. This polymorphism in the mother increased the severity of IUGR caused by maternal smoking (119). Thus, maternal genotype can affect the sensitivity of the fetus to tobacco smoke.
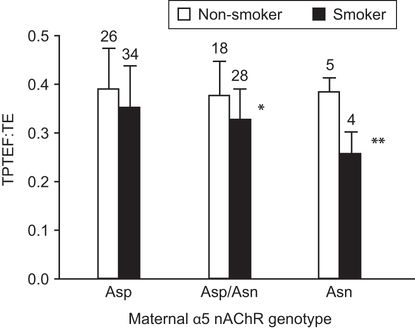
Infants whose mothers were homozygous for the risk allele of α5 single-nucleotide polymorphism (SNP) rs16969968 in which amino acid 398 of the α5 nAChR is changed from Asp to Asn showed the largest decrease in TPTEF:TE (ratio of time to peak tidal expiratory flow to expiratory time) if mothers smoked during pregnancy. Error bars show standard deviation. Asp = mothers homozygous for nonrisk allele (Asp/Asp), Asp/Asn = heterozygotes, Asn = mothers homozygous for risk allele (Asn/Asn). * p < .01, ** p < .001 compared to nonsmokers of same genotype by t-test.
Polymorphisms in the glutathione transferases (GST) (120), enzymes critical for antioxidant defenses, are examples of how the infant’s genotype makes the child more sensitive to effects of environment on lung health. Deletions in GST M1 increase the sensitivity of children with asthma to ozone (121). Infants with deletions in GST M1 and GST T1 are more sensitive to respiratory effects of maternal smoking during pregnancy (122,123).
In addition to genetic mechanisms, epigenetic mechanisms can influence environmental effects on lung development. This was demonstrated by Rehan et al. (124), who showed that exposure of mice to prenatal nicotine increased airway reactivity and increased DNA methylation. Increased airway reactivity and the alterations in DNA methylation were transmitted to subsequent generations even without continued nicotine exposure. Epigenetic changes also likely mediate the effects of maternal smoking on human lung development as well because maternal smoking changes DNA methylation in placenta, cord blood, and epithelial cells of the offspring (125,126).
Environmental Effects of Climate Change and Lung Development
While the potential for climate change to affect lung development and disease is speculative, climate change is likely to alter patterns of air pollution and allergens. It is therefore reasonable to speculate that changes related to global warming would affect lung development and disease. The American Thoracic Society (127) and the European Respiratory Society (128) have issued position papers outlining the potential effects of climate change on respiratory health and its potential effects on lung development. Higher temperatures may result in more air pollution greater energy use for air conditioning (127,128) and increased PM associated with forest fires that may be more common (129). Higher temperatures combined with increased energy needs may result in higher levels of ground-level ozone (130). Thus, increases in PM and ozone may occur with climate change and bring about adverse effects on lung development and respiratory systems.
In addition, environmental pollution and climate change are likely to increase pollen associated with higher temperatures and changing rain patterns (127,129,130). Increased temperatures and flooding may also be associated with increased mold (130). The combination of increased pollution and increased allergens (pollen and mold) has the potential for increasing asthma incidence.
Future Prospects
It is difficult to forecast the effect of environmental changes or the burden of lung disease in the future. Increasing population and climate change are likely to cause increased air pollution. Decreasing tobacco use and more use of less-polluting indoor stoves may decrease environmental pollution. The impact of E-cigarettes on human health is an evolving experience. It is hoped that data regarding its import will result in regulation that may minimize its impact in infant health. There will undoubtedly be more personalized-medicine approaches brought to bear on the potential effects of the environment. New genomic techniques, be it SNP analysis or direct DNA sequence analysis, will allow for the identification of individuals most at risk for the effects of smoking, air pollution, or allergic stimuli. Targeted efforts to lessening the environmental risk or specific therapeutic interventions, including in utero therapies, may moderate lung disease in the future.
References
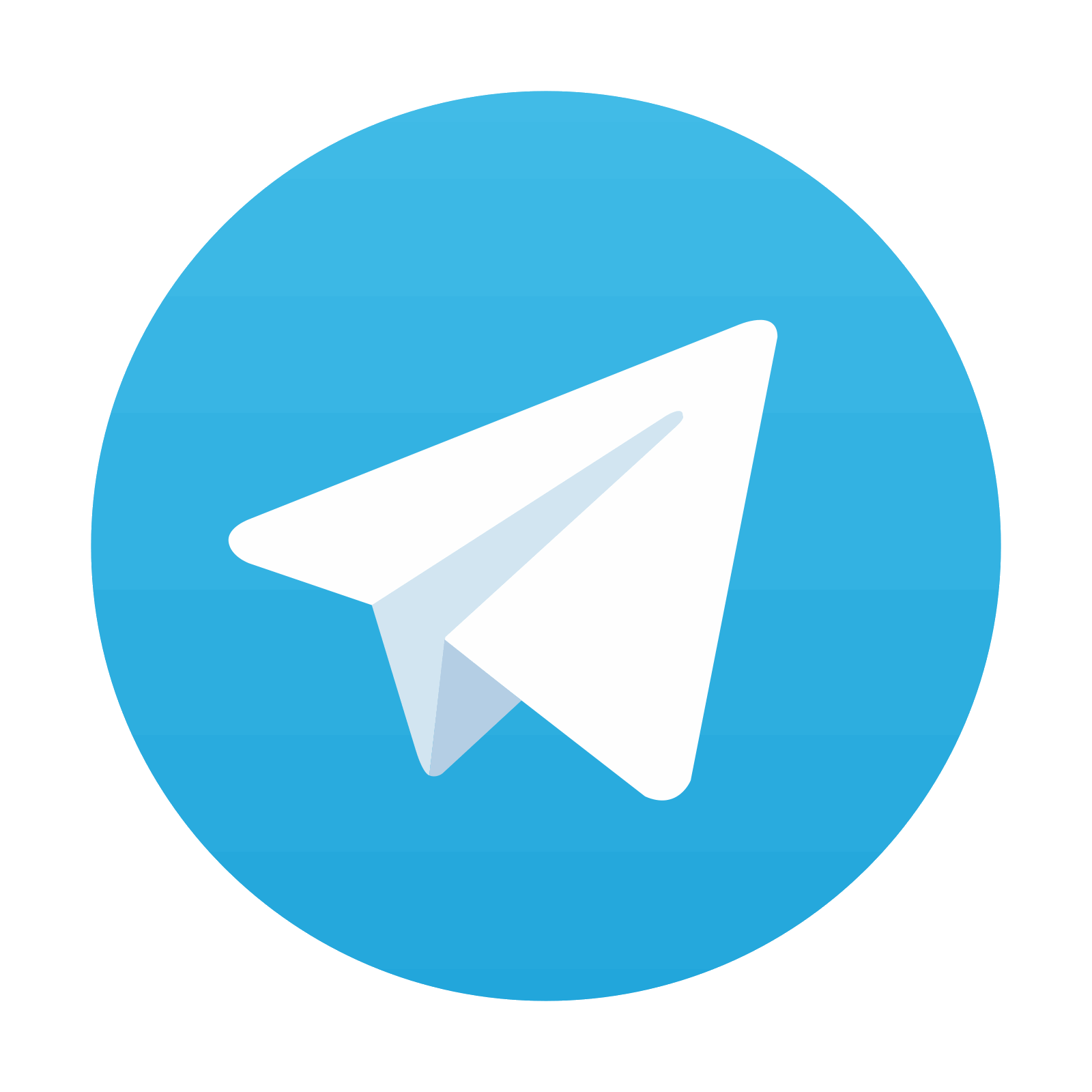
Stay updated, free articles. Join our Telegram channel
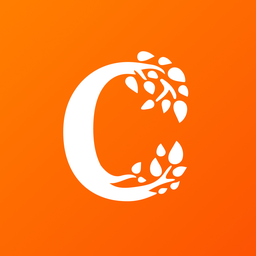
Full access? Get Clinical Tree
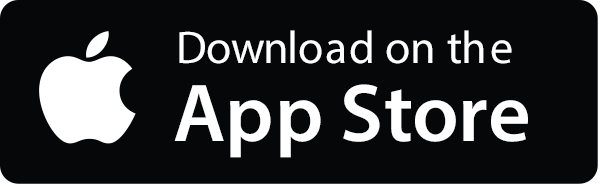
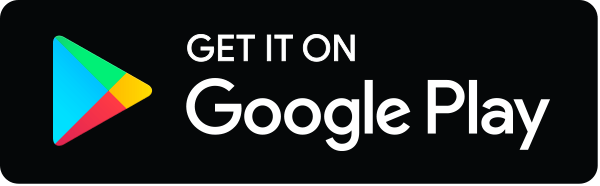