Although several investigations have demonstrated that prolonged aerobic exercise results in decreased left ventricular (LV) function, few have examined the impact of an acute bout of high-intensity exercise on right ventricular (RV) and LV systolic and diastolic function. Cardiac magnetic resonance imaging with tagging was used to study the impact of high-intensity interval exercise on biventricular function in 9 endurance-trained (ET; V o 2 max 69 ± 7 ml/kg/min) and 9 normally active (NA; V o 2 max 44 ± 9 ml/kg/min) men. Subjects underwent baseline cardiac magnetic resonance imaging assessments (pre) and then performed an average of 14 1-minute intervals at 97 ± 11% (NA) and 99 ± 6% (ET) of peak power output, separated by 2 minutes of recovery at 21 ± 6% (NA) and 21 ± 9% (ET) of peak power output. After exercise, 2 cardiac magnetic resonance imaging assessments (post 1 at 6.2 ± 2.6 minutes and post 2 at 38.4 ± 3.8 minutes) were completed. RV and LV ejection fractions, twist, basal and apical rotation rates, rate of untwisting, circumferential strain, and timings were examined. No significant change in RV and LV ejection fractions, twist, untwisting rate, or strain after exercise occurred in the NA group. In the ET group, RV ejection fraction (pre 56 ± 4%, post 1 54 ± 4%, post 2 54 ± 3%) and LV ejection fraction (pre 62 ± 4%, post 1 59 ± 4%, post 2 58 ± 4%) were decreased at post 1 and post 2, while untwisting rate, apical rotation rate, and circumferential strain were decreased at post 2 (all p values <0.05). In conclusion, biventricular systolic and diastolic dysfunction occurred after 14 minutes of high-intensity exercise in ET athletes, a phenomenon not observed in NA subjects.
Although numerous studies have examined cardiovascular function after prolonged submaximal exercise, the impact of brief high-intensity exercise is unknown. The aim of this study was to evaluate the effects of repeated bouts of maximal intensity exercise on biventricular systolic and diastolic function in endurance-trained (ET) and normally active (NA) subjects using cardiac magnetic resonance imaging. We hypothesized that high-intensity interval exercise would decrease right ventricular (RV) and left ventricular (LV) systolic and diastolic function in the 2 groups, with greater decreases in function occurring in NA subjects.
Methods
This study was approved by the University of Alberta Health Research Ethics Board (Biomedical Panel), and all participants provided written informed consent before the experiment. This investigation was conducted in accordance with the Declaration of Helsinki.
Nine healthy, NA men not participating in a regimented aerobic exercise program (V o 2 max <50 ml/kg/min) and 9 ET men who trained for >10 h/week (V o 2 max >60 ml/kg/min) and who regularly completed endurance and interval exercise were recruited for this study.
Participants reported to the Mazankowski Alberta Heart Institute on 2 occasions separated by a minimum of 48 hours. On day 1, subjects underwent basic anthropometric measurements and incremental cycle tests to exhaustion to determine V o 2 max. On day 2, subjects underwent baseline cardiac magnetic resonance imaging assessments. They then performed high-intensity interval exercise sessions on cycle ergometers, followed by 2 additional cardiac magnetic resonance imaging assessments.
The incremental exercise test was performed on an electronically braked cycle ergometer (Ergometrics er800s; Ergoline, Bitz, Germany) to assess V o 2 max and determine the exercise workloads for the second experimental day. The workload was increased by 20 W every 2 minutes until reaching the ventilatory threshold; thereafter, power output was increased by 20 W/min until exhaustion. Heart rate and expired gas analysis (Parvomedics, Salt Lake City, Utah) were obtained at rest and during exercise.
After a 15-minute self-selected warm-up, subjects were instructed to perform 15 1-minute workloads at 100% of the power output achieved at V o 2 max, separated by 2 minutes of active recovery. This protocol was chosen to provide a cardiac stimulus that was similar to interval training conducted by ET subjects, as well as activities performed by NA subjects (e.g., during a game of soccer or hockey). Average and peak power output and heart rate were recorded for each interval, and subjects drank water ad libitum.
Cardiac magnetic resonance imaging examinations were performed with a 1.5-T Siemens Sonata magnetic resonance imaging scanner (Siemens Medical Solutions, Erlangen, Germany) using a 5-element cardiac array for signal reception. Short-axis cines covering the RV and LV (10 to 12 slices, 8-mm slice thickness, 2-mm gap) were used to measure end-systolic volume, end-diastolic volume (EDV), stroke volume, and ejection fractions (echo time 1.5 ms, repetition time 3.0 ms, flip angle 60°, matrix size 256 × 192, field of view 380 mm, 30 reconstructed cardiac phases). E- and A-wave blood velocities (at the level of the mitral leaflet tips) and E′ tissue velocities (at the mitral annulus) were measured from short-axis through-plane phase-contrast cines (echo time 2.5 ms, repetition time 5.0 ms, flip angle 15°, slice thickness 8 mm, generalized autocalibrating partially parallel acquisition factor 2, velocity encoding strength 100 cm/s for the E/A ratio and 50 cm/s for E′). Phase-contrast studies had a temporal resolution of 29.5 ms for all subjects, interpolated to 50 phases for all subjects. Grid myocardial tagging was used to measure peak twist and untwisting rate and circumferential strain and strain rate (echo time 2.2 ms, repetition time 4.0 ms, flip angle 12°, 5 slices, slice thickness 8 mm, matrix size 192 × 128, temporal resolution 20 ms). Images were tagged with a delay of 200 ms after the electrocardiographic R wave to ensure tag persistence throughout diastole. Apical slices were always prescribed 1 cm from the true apex (as viewed on 2-, 3-, and 4-chamber images) with an 8-mm gap between additional short-axis slices, up to the level of the most base slice (in which myocardium can be seen in all cardiac phases). All image acquisitions were electrocardiographically gated and acquired during breath holds. Data were acquired in the order listed previously. Blood pressure (cuff sphygmomanometer) and heart rate (on electrocardiography) were monitored throughout acquisition.
Assessment of LV and RV volumes was performed by manual segmentation of short-axis cine images at end-diastole and end-systole (Argus; Siemens Medical Solutions). LV and RV EDV and end-systolic volume were calculated using the true method of disks, from which stroke volume and ejection fractions were determined. LV wall stress was calculated as 0.133 × P × R/2T × (1 + T/2R), where P is systolic blood pressure; R and T are the inner radius and wall thickness, respectively; and 0.133 is a conversion factor. LV mass was also normalized to body surface area .
Image processing and data analysis of phase-contrast and tagged images were performed using a custom designed software package. Among the methods used to assess LV twist, tagged cardiac magnetic resonance imaging is currently considered the reference standard. Tag analysis was fully automated, using an image-morphing algorithm to determine the spatial deformation field for the myocardium for each slice as a function of cardiac phase. The tag-processing software was developed and is used in the MATLAB (The MathWorks, Natick, Massachusetts) environment using an open-source algorithm for the image morphing calculations ( http://elastix.isi.uu.nl ). User input was limited to tracing the endocardium and epicardium at a single cardiac phase for each slice. Twist curves were calculated as the difference between the clockwise rotation at the base and the counterclockwise rotation at the apex, yielding a measure of the total basal-to-apical deformation. Peak twist was defined as the peak value on the curve, and peak untwisting rate was calculated as the minimum value of the time derivative of the twist curve directly after the time of peak twist. Circumferential strain was measured as the fractional change in circumferential length, averaged over the full width of the myocardium, between a given cardiac phase and a reference phase at diastasis, and the strain rate is the time derivative of this value. Circumferential values were averaged over the 5 short-axis slices. All parameters were plotted as a function of time from the QRS interval, and peak values were selected manually and stored with the times of the peak values. For temporal analysis, to account for individually dependent heart rates, the time sequence was normalized to the percentage duration of systole, with onset of the electrocardiographic QRS interval defining t = 0% and aortic valve closure defining end-systole, at which t = 100%. Values are provided in milliseconds as well. An untwisting performance metric was calculated by dividing the peak rate of untwisting by the peak twist, which normalizes the rate of untwisting to the preceding peak systolic twist and yields a potentially more diastolic specific measure of function. Images were analyzed by a single blinded technician.
A nonparametric test (Freidman’s analysis of variance ranks test) was used to make comparisons within groups, while between-group differences were calculated using the Mann-Whitney U test (Statistica; StatSoft, Ltd., Tulsa, Oklahoma). The change in LV and RV ejection fractions from before to after exercise (Δ scores) was correlated with age, V o 2 max, as well as Δ scores for heart rate and estimates of preload (EDV) and afterload (wall stress) using Pearson’s correlation coefficient. Data are presented as mean ± SD, and the significant level was set a priori at p <0.05.
Results
Participant characteristics are listed in Table 1 . No significant group differences were found for age, height, or body surface area. The NA group had significantly greater body mass and lower LV mass compared to the ET group. By study design, Vo 2 max was significantly lower in NA versus ET subjects.
Variable | NA (n = 9) | ET (n = 9) |
---|---|---|
Age (years) | 35 ± 7 | 29 ± 6 |
Height (cm) | 177 ± 7 | 180 ± 11 |
Body mass (kg) | 84 ± 6 ⁎ | 73 ± 9 |
Body surface area (m 2 ) | 2.0 ± 0.1 | 1.9 ± 0.2 |
V o 2 (ml/kg/min) | 44 ± 9 ⁎ | 69 ± 7 |
LV mass (g) | 152 ± 23 ⁎ | 173 ± 19 |
LV mass/body surface area 1.5 (g/m 2 ) | 53.2 ± 8.1 ⁎ | 65.5 ± 10.2 |
Table 2 summarizes the mean exercise performance data during the high-intensity interval exercise session. The average combined duration of all intervals was 14.0 ± 0.01 minutes. The average duration of the entire exercise session, including the warm-up, intervals, and recovery between intervals, was 58.7 ± 0.08 minutes. There was no change in body mass after exercise.
Variable | NA (n = 9) | ET (n = 9) |
---|---|---|
Number of intervals | 14 ± 2 | 14 ± 1 |
Interval heart rate (beats/min) | 169 ± 9 | 174 ± 7 |
% of maximal heart rate | 93 ± 4 | 93 ± 4 |
Recovery heart rate (beats/min) | 133 ± 13 | 135 ± 7 |
% of maximal heart rate | 73 ± 6 | 72 ± 4 |
Interval power output (W) | 291 ± 81 ⁎ | 402 ± 76 |
% of maximal power output (W) | 97 ± 11 | 99 ± 6 |
Recovery power output (W) | 66 ± 26 ⁎ | 95 ± 40 |
% of maximal power output | 21 ± 6 | 21 ± 9 |
The first postexercise (post 1) cardiac magnetic resonance imaging assessment began 6.2 ± 2.6 minutes after completion of the last interval, and the second assessment (post 2) began 38.4 ± 3.8 minutes after exercise. Table 3 lists the hemodynamic data. Heart rate at rest was significantly greater in the NA group compared to the ET group before exercise. There were no statistical differences in systolic, diastolic, or mean arterial blood pressures before exercise between NA and ET subjects. Compared with before exercise, there was a significant increase in heart rate and a significant decrease in wall stress at post 1 and post 2 in the 2 groups. There were no differences in end-systolic pressure/LV end-systolic volume after exercise in either group. Systolic blood pressure and mean arterial pressure were significantly decreased at post 2 in the 2 groups compared to before exercise. Preexercise LV and RV EDVs, end-systolic volumes, and stroke volumes were lower in NA compared to ET subjects (all p values >0.05). In the NA group, LV and RV EDVs and stroke volumes were significantly decreased at post 1 and post 2 compared to before exercise, while LV and RV end-systolic volumes were significantly decreased at post 1 only. In the ET group, there were no differences after exercise in LV or RV end-systolic volume, while LV and RV EDVs were significantly lower at post 1 only. LV and RV ejection fractions decreased significantly at post 1 and post 2 in the ET group, whereas no change in LV or RV ejection fraction occurred in the NA group after exercise ( Figure 1 ). The LV E/A ratio was decreased at post 2 in the 2 groups (NA: pre 1.9 ± 0.5 vs post 2 1.2 ± 0.3, p <0.05; ET: pre 2.1 ± 0.5 vs post 2 1.6 ± 0.5, p <0.05). After exercise, LV E′ was significantly decreased at post 2 in the 2 groups. There was no change after exercise in LV S′, RV S′, or RV E′ in the ET group, while RV E′ was significantly increased in the NA group at post 1. There were no correlations between changes in LV ejection fraction and age, V o 2 max, exercise power output normalized to body mass, LV EDV, or heart rate. Similarly, no significant correlations were found between changes in RV ejection fraction and age, exercise power output normalized to body mass, V o 2 max, RV EDV, or heart rate.
Variable | Pre | Post 1 | Post 2 | |||
---|---|---|---|---|---|---|
NA | ET | NA | ET | NA | ET | |
Heart rate (beats/min) | 65 ± 6 † | 55 ± 6 | 96 ± 13 ⁎ | 87 ± 8 ⁎ | 84 ± 13 ⁎ | 73 ± 8 ⁎ |
Systolic blood pressure (mm Hg) | 123 ± 9 | 121 ± 12 | 120 ± 12 | 118 ± 6 | 115 ± 9 ⁎ | 109 ± 7 ⁎ |
Diastolic blood pressure (mm Hg) | 74 ± 11 | 71 ± 7 | 75 ± 7 | 69 ± 6 | 72 ± 8 | 67 ± 8 |
Mean arterial pressure (mm Hg) | 90 ± 9 | 88 ± 8 | 90 ± 7 | 85 ± 5 | 86 ± 8 ⁎ | 81 ± 6 ⁎ |
Left ventricular wall stress | 85 ± 15 | 89 ± 20 | 70 ± 16 ⁎ | 82 ± 20 ⁎ | 67 ± 13 ⁎ | 76 ± 18 ⁎ |
SBP/LV end-systolic volume (mm Hg/ml) | 1.9 ± 0.4 | 1.6 ± 0.4 | 2.3 ± 0.7 | 1.7 ± 0.5 | 1.9 ± 0.4 | 1.4 ± 0.4 |
There were no significant differences between groups for cardiac magnetic resonance imaging tagging data before exercise. After exercise, there were no significant changes in twist, untwisting rate, apical or basal rotation rates, or circumferential strain in the NA group ( Figure 1 ). In the ET group, there was no change in twist or basal rotation rate after exercise; however, untwisting rate, apical rotation rate, and circumferential strain were significantly decreased at post 2 ( Figure 1 ). Additionally, the untwisting performance metric (i.e., untwisting rate/twist) was significantly decreased at post 1 and post 2 in the ET group, but no differences after exercise were observed in the NA group. Twist was not related to LV ejection fraction, end-systolic volume, or EDV at any time point. Untwisting rate was significantly related to twist at all time points for all subjects (pre r = 0.81, post 1 r = 0.92, post 2 = 0.79; p <0.05).
The timing of peak twist and peak untwisting rate were significantly later after exercise in the ET group, with no differences in the NA group ( Table 4 ). It has previously been shown that the onset of increase in LV volume occurs simultaneously with the onset of relaxation in the circumferential plane. Consequently, peak circumferential strain rate may be used to infer LV filling. There was a significant reduction in the interval between the time of peak untwisting to the time of peak circumferential strain rate (LV filling) at post 1 and post 2 in the ET group, with no change after exercise in the NA group. Given that there is normally a distinct separation between untwisting and filling in healthy hearts (i.e., relaxation largely precedes peak filling), the reduced time between untwisting and filling after exercise in the ET group likely reflects impaired diastolic function. Figure 2 compares the reduction in this time interval from baseline to post 2 with the corresponding reduction in the rate of untwisting in the ET group, demonstrating a good correlation between changes in these parameters. The time between peak twist and peak circumferential strain increased significantly in all subjects at post 1, but only in the ET group at post 2. This reflects the larger delay in the time of peak twist in ET subjects after high-intensity exercise ( Table 4 ).
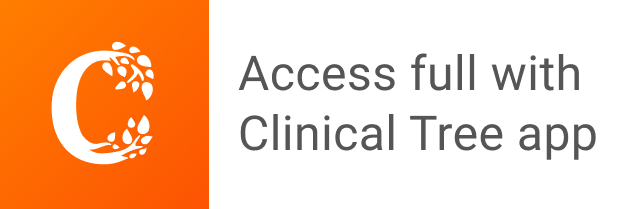