Introduction
Diving and work in compressed air have produced occupational exposure to hazardous environments for over 100 years. In the past 60 years, diving has become a recreation for millions of individuals throughout the world. Any physician may encounter a recreational diver for an illness related to a diving exposure or for medical clearance for diving. Occasionally a physician may be confronted with an acute medical emergency related to diving exposure, such as decompression sickness, arterial gas embolism, or drowning. This chapter provides a basis for initiating treatment, seeking consultation, or furthering education in this interesting area of environmental medicine.
Increased Pressure
Underwater exposure results in increasing pressure directly proportional to depth ( Table 78-1 ). There is also exposure to pressure in hyperbaric chambers, pressurized tunnels and caissons used for underwater construction, and underwater habitats. As depth increases, the diver breathes gas of increased density using breathing equipment that provides oxygen and allows for elimination of carbon dioxide. Increased depth and pressure also lead to decreases in gas volume and an increased amount of gas dissolved in body tissues. The terms atmosphere (ATM) and atmosphere absolute (ATA) both refer to pressure; ATM, however, can be used as a relative term (33 feet of water depth is equivalent to 1 ATM of water), whereas ATA is always used for absolute pressure (33 feet of water depth is equivalent to 2 ATA). ATA is the term used in this chapter and is always used for equations, such as Boyle’s law.
Feet | ATA | mm Hg | psi | |
---|---|---|---|---|
Altitude above sea level | 12,000 | 0.636 | 483 | 9.3 |
8000 | 0.742 | 564 | 10.9 | |
4000 | 0.863 | 656 | 12.7 | |
Sea level | 0 | 1 | 760 | 14.7 |
Depth in seawater | 33 | 2 | 1520 | 29.4 |
66 | 3 | 2280 | 44.1 | |
99 | 4 | 3040 | 58.8 | |
132 | 5 | 3800 | 73.5 |
Water Immersion
Intrathoracic blood volume increases with water immersion. Increased hydrostatic pressure during immersion prevents blood from pooling in the peripheral veins, increases transdiaphragmatic pressure, and increases venous return. The magnitude of the increase in intrathoracic blood volume during immersion with the head above water has been estimated to be 700 mL. The central blood shifts result in an increase in cardiac output and an increase in central venous pressure. The increase in intrathoracic blood volume causes a diuresis because of release of natriuretic hormones and suppression of antidiuretic hormone.
Thermal Exposure
Most diving takes place in water colder than skin temperature, and the diver loses heat throughout the dive. Hypothermia happens rapidly in the absence of protective garments even in relatively warm water (e.g., 22° to 23° C). Cold stress evokes thermogenic responses, reflected by a rise in oxygen consumption ( ) upon exposure to cold water to generate additional body heat and minimize core temperature change. Cold water diving also leads to peripheral vasoconstriction; the magnitude of the vasoconstriction is dependent on body core temperature, which, in turn, is affected by the amount of thermal protection and the water temperature. Heat loss is inhibited in divers doing moderate work in water above 30° C, and, in this situation, hyperthermia can develop.
Energy Needs for Diving
A scuba ( self-contained underwater breathing apparatus ) diver swimming underwater at a speed of 1.0 knot (about 100 feet/min or 1.15 miles per hour or 1.85 km per mile) consumes about 25 mL of oxygen per kilogram per minute ( Fig. 78-1 ). A diver with a maximum of 40 mL/kg per minute would tolerate swimming at 1.3 knots for a few minutes but would become extremely tachypneic to compensate for lactate production. For a diver with a maximum
of 40 mL/kg per minute, sustainable swimming speed, working at 50% of maximum capacity, would be about 0.9 knots or 90 feet/min, whereas the diver with a maximum
of 25 mL/kg per minute would be able to sustain a swimming speed of only approximately 0.55 knots or 55 feet/min. Safety considerations suggest that the sport diver should be able to tolerate a sustained workload of about 20 mL/kg per minute (50% of maximum). Poorly conditioned divers can experience severe dyspnea under even mildly stressful conditions that exceed their anaerobic threshold.

Equipment
Open-Circuit Scuba
The most commonly used breathing equipment in diving is the open-circuit scuba. This equipment ( Fig. 78-2 ) consists of a metal cylinder containing compressed air connected to a pressure regulator that lowers pressure to ambient pressure. This device delivers ambient-pressure air only when inhalation is initiated and allows flow that matches the minute volume of the diver. Expired air is released directly into the surrounding water. A typical scuba cylinder can supply approximately 2100 L of air at the surface (1 ATA). This volume is reduced in direct proportion to ambient pressure. For example, at a depth of 66 feet of seawater or 3 ATA pressure, the effective volume of air is decreased to 700 L. With a minute ventilation of 20 L/min, this air supply would last 105 minutes at the surface but only 35 minutes at 66 feet of seawater. Open-circuit scuba is usually limited to depths above 200 feet because of limited air supply, nitrogen narcosis, and oxygen toxicity.

Closed-Circuit Rebreather Scuba
The self-contained rebreather uses a carbon dioxide absorbent to remove exhaled carbon dioxide and replenishes only the oxygen used. Inert gas is conserved by recycling the exhaled gas through the carbon dioxide absorbent and then adding oxygen before the gas is rebreathed (see Fig. 78-2 ). Little gas is released into the surrounding water, oxygen can be carried in volumes adequate for several hours of exposure, and gas consumption is independent of depth. Although use of closed-circuit scuba in the past was confined to commercial and military divers, such systems now are becoming popular among recreational divers.
Surface-Supplied Equipment
Commercial diving often employs this form of equipment. The diver breathes compressed air or other gas mixtures pumped from the surface to the helmet. The diving helmet is attached at the collar to a diving suit so that air flows from the helmet into the suit to maintain an air layer for thermal protection. Modern systems contain a demand mask built into the helmet.
Disorders Related to Diving: Nomenclature
Golding and coworkers described disorders related to supersaturation of inert gases in tissues with subsequent bubble formation as decompression sickness (DCS). They described a systemic form of DCS that involves the central nervous system, the lungs, and the circulation (“serious,” type II) and a nonsystemic (peripheral) form that involves the skin, bones, and joints (“minor,” type I). Arterial gas embolism is named separately based on its relationship to pulmonary barotrauma. Francis and Smith suggested the term decompression illness for these two disorders because they can be clinically difficult to separate and require similar therapy.
Pressure Effects: Boyle’s Law
Relation of Gas Volume to Depth
Boyle’s law states that, if the temperature of a fixed mass of an ideal gas is kept constant, volume and pressure are inversely related. Consequently, when the pressure is doubled, the volume is reduced to one half of the original volume. Because the gas volume is proportional to the absolute pressure, the volume change from the surface to 33 feet of seawater (from 1 to 2 ATA) is greater than the change from 33 to 66 feet (from 2 to 3 ATA).
Barotrauma
With increased pressure, volume in the lungs, middle ear, paranasal sinuses, and gastrointestinal tract are reduced. Displacement of tissues into the diminishing volume of these spaces may cause tissue injury and dysfunction of the organ involved. Barotrauma can affect a paranasal sinus with an occluded orifice, a residual air pocket left between a tooth filling and the base of the tooth, or the air space within a diving mask.
Pulmonary Barotrauma and Arterial Gas Embolism
The gas a diver breathes is pressurized to the ambient pressure so that pressure gradients from the breathing supply to the airways are not altered as the diver descends. Behnke and Polak and Adams described lung barotrauma in ascending divers due to inadequate exhalation during ascent and overexpansion of the lungs. Later studies provided further insight into mechanisms and prevention of pulmonary barotrauma. After breathing compressed air, persons who ascend to the surface from depths as shallow as 4 feet can experience pulmonary barotrauma.
Pathophysiology.
Under experimental conditions, transpulmonary pressures (i.e., the difference between intratracheal and intrapleural pressures) of 95 to 110 cm H 2 O are sufficient to disrupt the pulmonary parenchyma and force gas into the interstitium. Extra-alveolar gas will migrate through perivascular sheaths to cause mediastinal emphysema and pneumothorax. Gas can also dissect into the retroperitoneum and into the subcutaneous tissues of the neck. Extra-alveolar gas can pass into ruptured blood vessels, travel to the left side of the heart, and enter the arterial circulation as gaseous emboli. The dissemination of gas bubbles throughout the arterial circulation causes injury to other organ systems and to skeletal muscle, which is evident by a rise in serum creatine kinase level.
Pulmonary barotrauma can be seen in divers who would not be considered at risk for lung overpressure. Occult lung disease may contribute to unexplained barotrauma and cerebral air embolism. Epidemiologic studies have not demonstrated a significant relationship between asthma and an increased risk for pulmonary barotrauma.
Clinical Manifestations of Arterial Gas Embolism.
The brain is commonly involved. Within minutes of surfacing, the diver can experience loss of consciousness, hemiplegia, stupor, and confusion. Seizures, vertigo, visual disturbances, sensory changes, headache, and circulatory collapse are common. Most individuals fully recover if they are promptly recompressed.
When they lose consciousness in the water, victims of arterial gas embolism frequently drown. Chest radiographs ( Fig.78-3 ) may show a diffuse lung edema pattern. About 5% of patients immediately develop apnea, unconsciousness, and cardiac arrest. This catastrophic course results from filling of the heart and great vessels with air. Many of these individuals are unresponsive to cardiopulmonary resuscitation and advanced life support measures. A report of 31 patients with cerebral air embolism from diving included the following findings: 25% demonstrated pneumomediastinum; 10%, subcutaneous emphysema; 6%, pneumocardium; 3%, pneumoperitoneum; and 3%, pneumothorax. Fifty-two percent had pulmonary opacities indicating associated drowning.

Mediastinal emphysema is generally associated with mild substernal pain that may be exacerbated by inspiration, coughing, or swallowing. Unless massive, this condition is not usually associated with circulatory compromise. On physical examination a crunching sound synchronous with cardiac action may be auscultated (the Hamman sign). The chest radiograph confirms the diagnosis. No treatment is usually necessary.
Subcutaneous emphysema causes swelling and crepitus in the base of the neck and supraclavicular fossa, sore throat, hoarseness, and dysphagia. Radiographs may be helpful in detecting subtle cases, but computed tomography (CT) scans are more sensitive and can be useful to confirm the diagnosis of barotrauma in doubtful cases. Extra-alveolar gas that ruptures into the pleural space will cause a pneumothorax. Laboratory evaluation may show an elevated hematocrit level and elevation of several serum enzyme levels. Treatment for arterial gas embolism requires recompression in a hyperbaric chamber (see later).
Middle Ear Barotrauma
Middle ear barotrauma is the most common diving-related disorder encountered in divers. The middle ear undergoes barotrauma when the eustachian tube is blocked during descent and the middle ear space cannot equilibrate with the increasing ambient pressure. The tympanic membrane is displaced inward and may rupture. The middle ear may fill with blood from engorged mucous membranes. Infection and hearing loss are complications. Symptoms during descent include pain in the affected ear that increases with depth. Relief of pain without proper equalization of the middle ear pressure usually indicates that the tympanic membrane has ruptured. Cold water entering the middle ear when the tympanic membrane ruptures may cause vertigo because of unilateral vestibular stimulation. Late complications include bacterial otitis media, serous otitis media, and chronic tympanic membrane perforation. In rare cases of middle ear barotrauma, the facial nerve is injured by the increased pressure and a temporary facial paralysis results. A modified Valsalva maneuver is commonly used to equilibrate middle ear pressure. Because middle ear barotrauma causes edema and hemorrhage in the middle ear, equalization is usually impossible to achieve until healing is complete. The presence of middle ear barotrauma usually prohibits diving until it is resolved.
Alternobaric Vertigo.
Vertigo may develop on ascent when the reduction of middle ear pressure is not uniform in both ears. The pressure imbalance causes differential stimulation of the labyrinths, resulting in what is called alternobaric vertigo. The sensation of vertigo may persist for 1 to 2 hours after diving and gradually disappears without therapy. Symptoms are similar to labyrinthitis and can include nausea, vomiting, and generalized malaise. Some subjects may be particularly susceptible to alternobaric vertigo if they have had previous injury or infection of the labyrinths. In susceptible individuals, use of moderate doses of antihistamines or decongestants may prevent symptoms. The disorder must be differentiated from vestibular DCS, which is usually associated with deeper, prolonged diving.
Inner Ear Barotrauma
Inner ear barotrauma may develop on descent in divers who perform a forceful Valsalva maneuver to equalize middle ear pressure. When the eustachian tube is blocked, middle ear pressure becomes progressively more negative relative to ambient pressure. When a Valsalva maneuver is then performed, intrathoracic pressure, central venous pressure, spinal fluid pressure, and inner ear pressure rise above ambient pressure, thereby increasing the gradient between the inner ear perilymph and the middle ear. The round or oval window can rupture, and perilymph can then leak from the inner ear to the middle ear.
Symptoms include vertigo, nausea, vomiting, tinnitus, and loss of hearing on the affected side. Severity may vary, and some divers complain of hearing loss, tinnitus, or vertigo only after diving.
Treatment varies from conservative therapy to surgical repair of the round or oval window. Vertigo and nausea can be treated with benzodiazepine medications. Tinnitus and reduced hearing may become chronic, particularly if no treatment is provided. Divers who exhibit clinical evidence of inner ear barotrauma with intact round and oval windows may have a pressure injury to the organ of Corti and the vestibular system.
Inner ear DCS may be seen within 2 hours of surfacing and may include vertigo and hearing loss. The mechanism is poorly understood but may involve formation of bubbles in the inner ear or embolism of systemic bubbles. Divers with inner ear DCS have been reported to have a higher prevalence of patent foramen ovale (PFO), a condition that increases risk for right to left shunts of air bubbles and thus of decompression events; such an association suggests that the inner ear DCS may also result from air emboli. The proper therapy for inner ear DCS is hyperbaric recompression.
Sinus Barotrauma
When a sinus orifice is occluded, pressure within the sinus becomes negative with respect to ambient pressure, and mucosal blood vessels become engorged and eventually rupture. Pain over the affected sinus during descent and epistaxis on ascent are usual symptoms. Headache following a dive may indicate sphenoid sinus barotrauma. Treatment includes decongestants and maneuvers to drain the affected sinus. Persistence of blood in the sinus may result in bacterial sinusitis. Prevention is accomplished by avoiding diving with congestion of the nasopharynx and prudent use of decongestants. If a maxillary sinus orifice is occluded, the maxillary branch of the trigeminal nerve may be compressed during ascent and result in infraorbital paresthesias that usually resolve in 2 to 3 hours.
Less Common Forms of Barotrauma
Facial barotrauma (mask squeeze) happens in the area of distribution of the diving mask. Facial edema, ecchymoses, and conjunctival hemorrhages can be noted after diving. Retro-orbital hematoma and diplopia have been described as complications. The disorder is self-limiting; no treatment is needed.
Tooth barotrauma leading to severe toothache results when air pockets under fillings or in areas of decay become compressed on descent. Careful dental work prevents this disorder.
Gastrointestinal barotrauma results when air enters the stomach during diving due to faulty breathing apparatus or by air swallowing. On ascent the expanding air will distend the stomach or intestine. Gastric distention can occlude the esophageal-gastric junction and prevent eructation. Distention of the stomach may cause stomach rupture and pneumoperitoneum. The diver experiences abdominal pain, which increases during ascent. Treatment requires surgical repair of the ruptured viscus. Divers with previous gastric surgery may be prone to gastric air trapping.
Dissolved Inert Gas Effects: Henry’s Law
Inert Gas Kinetics
Gases dissolve in tissues, fats, and water according to Henry’s law: Q = c • P, where c, a solubility coefficient, and P, the partial pressure of the gas, determine the quantity (Q) of dissolved inert gas. Increased ambient pressure increases dissolved gas concentration in the tissues. The partial pressure of the gas and the solubility of the gas in the specific tissue ( Table 78-2 ) determine dissolved gas content. Although Henry’s law determines the amount of gas in the tissue, there is a finite time required for equilibrium to be achieved. Factors that affect the rate of entry include blood flow and the rate of diffusion of gases into the tissue. Tissue gas concentration follows an asymptotic curve in which the tissue gas concentration approaches the maximum concentration for the given pressure after time has elapsed. Similar kinetics control the washout of inert gas from tissues when ambient pressure is reduced. Different body compartments have different gas exchange characteristics.
Gas | Molecular Weight | Lipid Solubility † | Water Solubility | Narcotic Potential ‡ |
---|---|---|---|---|
Helium | 4 | 0.015 | 0.009 | 0.23 |
Neon | 20 | 0.019 | 0.009 | 0.28 |
Hydrogen | 2 | 0.036 | 0.018 | 0.55 |
Nitrogen | 28 | 0.067 | 0.013 | 1.00 |
Argon | 40 | 0.140 | 0.026 | 2.32 |
* Solubility of the various gases in lipid is related to their narcotic potential. Helium is the least, and argon the most, narcotic gas in the list.
† Expressed as gas volume/solute volume at 1 bar.
Because most diving is of short duration (i.e., minutes to hours) and shallow (i.e., in depths shallower than 200 feet), only a few tissue compartments reach equilibrium based on Henry’s law. Divers can return to the surface from these short-duration dives by following a schedule of ascent based on depth and time. When diving exposure is long enough, all tissues reach equilibrium at the new ambient pressure and are fully saturated with inert gas. Divers can spend prolonged periods (weeks) under pressure, with all tissues saturated at the increased pressure, without serious physiologic changes.
Inert Gas Supersaturation in Tissues
When the diver ascends after breathing pressurized gas, tissues become supersaturated with gas. When the degree of supersaturation becomes excessive, dissolved gas will leave solution and form free gas. Recent studies suggest that blood microparticles may act as a nidus for bubble formation in blood.
Decompression Sickness
Excess supersaturation causes dissolved gases to change phase to the gaseous form. Expansion of gases in blood and tissues on ascent results in damage and dysfunction of tissues and organs and venous gas embolism to the lungs. DCS is the disorder caused by damage to organs and tissues as a result of free gas production.
Bert first described the pathophysiology of DCS. Subsequent investigators in the early twentieth century concluded from autopsies on divers and caisson workers that DCS is caused by free gas in blood and tissues. Based on free gas volume and location, they were able to explain the variety and severity of the symptoms. Paralysis resulted from free gas in the spinal cord, cerebral dysfunction was thought to result from free gas in the brain, and dyspnea was associated with free gas in the pulmonary circulation. Muscle and joint pain may be due to free gas in ligaments, fascia, periosteum, marrow, or nerve sheaths.
Hallenbeck and colleagues described effects of free gas in blood and tissues that were not caused by mechanical obstruction. Subsequent studies identified clotting and platelet activation, intravascular coagulation, plasma leakage from the intravascular space, hemoconcentration, and hypovolemia as manifestations of surface effects of the bubbles. Venous gas emboli are commonly present before overt symptoms of DCS appear. Free gas and tissue injury result in activation of the inflammatory cascade. The inflammatory response causes fluid to leak into the interstitial tissues of the systemic and pulmonary vascular beds.
Factors Affecting Risk for Decompression Sickness
The use of ultrasonography for intravascular bubble detection has provided insight into the presence of bubbles following diving. Many divers demonstrate venous gas emboli but no manifestations of DCS. The concept of a threshold or dose-response effect has been postulated, wherein a certain volume of free gas is needed to produce the clinical disorder and lesser amounts are asymptomatic. Venous bubbles in asymptomatic divers and aviators are associated with increased risk for developing DCS after diving or altitude exposure. Exercise and temperature during decompression are considered to be risk factors for DCS. Breath-hold divers can develop DCS from frequent repetitive dives.
Symptoms can be prevented by keeping the degree of supersaturation below a certain level. The concept of a critical pressure ratio is the basis for most decompression tables used to prevent DCS. The body can be considered as a set of tissue compartments with different rates of gas uptake and elimination. Although these tissues do not represent discrete anatomic structures, they provide a convenient means for understanding the kinetics of inert gas exchange. In most decompression schedules, stops during ascent are selected to avoid excess supersaturation in tissues with specific gas-exchange rates ( Fig. 78-4 ). Decompression procedures are well defined for air and other mixtures of nitrogen and oxygen; helium and oxygen; nitrogen, helium, and oxygen (trimix); hydrogen and oxygen; and a few rare earth gases such as argon and neon.

Patent Foramen Ovale.
Moon and coworkers reported 30 patients with a history of DCS who were studied with echocardiography for identification of a PFO. Sixty-one percent of 18 patients with serious DCS had shunting, whereas a 25% prevalence was seen in normal volunteers. Wilmshurst and associates identified a single patient with paradoxical gas embolism through an atrial septal defect and suggested that the atrial septal defect augmented symptoms of DCS. Moon and coworkers evaluated 90 divers with previous DCS who were studied using echocardiography to detect right-to-left shunting through a PFO. Fifty-nine of 90 had experienced serious decompression symptoms, whereas 31 had experienced pain only or mild symptoms.
In the presence of a PFO, cerebral gas embolism can be caused by venous bubbles transiting a PFO. Germonpré and associates found that divers with cerebral DCS have a high incidence of large PFO compared to control subjects. Billinger and colleagues demonstrated an increase in magnetic resonance imaging–detected brain objects in divers with a PFO. Honek and colleagues demonstrated that a large PFO could increase risk of DCS in divers with exposures that resulted in significant venous gas emboli. The presence of a PFO may be associated with a twofold to fourfold increase in the risk for DCS. The high prevalence of PFO in the population and the very low incidence of DCS suggest that a PFO can play only a minor role in the pathophysiology of DCS. There is no current need to close a PFO as a prophylactic measure.
Age.
A 10-year study by the U.S. Air Force on flight exposures ranging from 1500 to 30,000 feet shows a threefold increase in susceptibility to altitude DCS in aviators 42 years of age and older compared with those 18 to 21 years of age. Carturan and colleagues found a correlation between bubble formation, increased age, and decreased physical condition in sport divers. Klingmann and coworkers reported an increased incidence of DCS in novice divers compared to more experienced divers. Blatteau and associates reported that age and depth of dive exposure increased DCS risk.
Relation to Altitude.
Exposure to altitudes above 18,000 feet (0.5 ATA) may result in free gas formation in tissues because of supersaturation of inert gases dissolved at atmospheric pressure. Divers can develop free gas in tissues when going to higher altitude after diving even though they follow established protocols for ascent from depth to the surface. It is common for sport divers to fly in commercial aircraft (about 8000 feet equivalent altitude) shortly after diving.
Clinical Manifestations
Compared to sport and military divers, commercial divers have the highest incidence of DCS. Musculoskeletal DCS (type I) is the most common form. The incidence of DCS is about 1 in 5000 dives for the sport diver.
DCS can mimic a variety of other disorders ( Table 78-3 ). Free gas entering the venous system will cause varying degrees of pulmonary vascular obstruction. A classic syndrome (“chokes”) manifested by chest pain, dyspnea, and cough is described. DCS is often associated with free gas in the blood and tissue injury that activates an inflammatory process, with damage to vascular endothelium, microvascular occlusion, and focal regions of tissue ischemia. A common manifestation of DCS in divers is spinal cord dysfunction, usually at levels below the diaphragm. Symptoms include paresthesias, muscle weakness, paralysis of the lower extremities, bowel or bladder incontinence, urinary retention, and sexual impotence. A sudden ascent from deep depth (blowup) can cause a massive DCS syndrome with both cerebral and spinal neurologic symptoms, unconsciousness, hypovolemic shock, pulmonary edema, and a high mortality rate. A rare but important symptom of serious (type II) DCS is sudden acute neurologic hearing loss and vestibular dysfunction. DCS of this type usually follows deep, prolonged diving exposures and, if untreated, can result in permanent deafness.
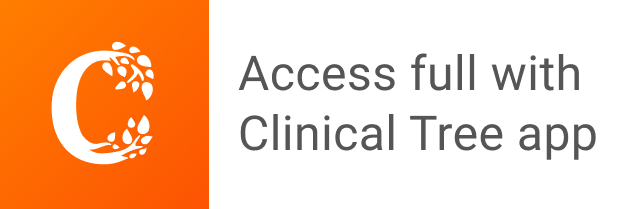