Diffusion, Chemical Reactions, and Diffusing Capacity
Uptake of oxygen and excretion of carbon dioxide require rapid, efficient exchange in the lung. The quantities of exchanged gases are staggering. For example, a 1800-calorie diet requires absorption of 375 L of oxygen per day, as well as excretion of a slightly smaller volume of carbon dioxide. Because blood remains in the pulmonary capillary bed for a limited time, the process of exchange must be accomplished in less than 0.75 second at rest and 0.5 second during exercise. This rapid, high-volume exchange occurs efficiently despite numerous interacting processes of diffusion and chemical reaction that occur in the lung. The rates of these processes are not only affected by intrinsic characteristics of blood but also determined by a host of other factors, including inspired oxygen fraction, alveolar gas tensions, cardiac output, and metabolic activity. The ease of exchange of respiratory gases belies the complexity of the overall process.
DIFFUSION
The concentration (C) of a gas dissolved in fluid depends upon its partial pressure (P) and solubility (α)
Gases diffuse from a higher to a lower partial pressure, not necessarily from a higher to a lower concentration. This fact is especially pertinent when a gas diffuses between two phases, as occurs when O2 and CO2 are exchanged between alveolar gas and blood. For example, dissolved CO2 diffuses down a partial pressure gradient from blood (46 mm Hg) into the alveolus (40 mm Hg), even though its actual concentration (millimoles of molecular CO2 per liter of gas or blood) is greater in alveolar gas (2.5) than it is in venous blood (1.4).
INFLUENCE OF PHYSICAL PROPERTIES
The rate of a gas diffusing through an aqueous membrane such as that separating alveolar gas and capillary blood is influenced by five factors. The rate is directly proportional to the surface area of the membrane, but inversely proportional to the thickness of the membrane. The rate increases in direct proportion to the difference in gas pressure between alveolar gas and capillary blood, and the diffusion and solubility coefficients of the gas in the membrane.
The diffusion coefficient of a gas in the alveolar–capillary membrane is largely a function of the size of the gas molecule, which is inversely proportional to the square root of its molecular weight (MW). Oxygen (MW 32) has a slightly greater diffusion coefficient than carbon dioxide (MW 44) in the alveolar membrane. However, the solubility of CO2 in water, the major component of tissue composing the membrane, is much greater than the solubility of O2. This difference far outweighs the effect of the slightly smaller size of the oxygen molecule. Thus, the rate of CO2 transfer across the alveolar membrane is approximately 20 times greater than that of O2 when both gases diffuse under the same partial pressure gradient. As a result, a much greater PO2 gradient across the membrane is required to maintain O2 transfer equal to that of CO2.
On the other hand, the rate of carbon monoxide (CO) transfer is very similar to that of oxygen when both gases diffuse across the alveolar–capillary membrane under the same partial pressure gradient. CO (MW 28) is a slightly smaller molecule than oxygen so its diffusion coefficient is slightly greater. This diffusive advantage is offset by a slightly lower aqueous solubility of CO compared to oxygen. As a result, CO and O2 transfer across the membrane have approximately equal rates at the same transmembrane partial pressure gradient.
The rate of diffusion is affected by the viscosity of the medium through which the diffusion occurs. Diffusion of a gas in air occurs at a rate that is four orders of magnitude greater than diffusion in water. Diffusion coefficients in tissues are only moderately less than those in water, since most tissues are composed primarily of water. The interior of the erythrocyte is an exception to this general rule. As a consequence of the high concentration of hemoglobin inside the red cell, the viscosity of the cell contents is substantially greater than that of water. This greater viscosity reduces the diffusion coefficient for oxygen to one-third of its aqueous coefficient. The combination of increased viscosity and the large size of the hemoglobin molecule decreases the diffusion coefficient of hemoglobin within the red cell to less than 10% of its diffusion coefficient in a dilute aqueous solution. As a result, significant diffusion gradients are thought to exist within the red cell even though the distance between the cell membrane and the innermost portion of the cell is only a few microns.
EFFECT OF DIFFERENT CAPACITANCES
The alveolar–capillary membrane provides a barrier to diffusion of gases between the alveoli and the capillaries. The rate of approach to diffusion equilibrium of a gas in the lung is dependent on the capacitances of the gas in the alveoli and blood relative to its solubility in the alveolar–capillary membrane. Normal ventilation of alveoli results in a large reservoir of oxygen with a pressure of ∼100 mm Hg to promote diffusive transfer across the alveolar–capillary membrane. The ability of hemoglobin to bind O2 increases the oxygen capacity of blood by two orders of magnitude compared with that of the alveolar–capillary membrane. This large capacitance for oxygen in blood requires substantial oxygen transfer across the membrane to reach diffusion equilibrium. Because the solubility of oxygen in the membrane is small relative to the large capacitances in alveolar gas and blood, oxygen exchange across the membrane requires 0.2 to 0.4 second to reach equilibrium.1 Fortunately, this delay in reaching equilibrium is less than the average of 0.75 second that blood remains in the pulmonary capillary bed. These same conditions are present during carbon monoxide transfer. The large capacitance and the low aqueous solubility of CO lead to similar impediments in gas exchange.
In contrast to oxygen and carbon monoxide, the solubility of carbon dioxide in the membrane is sufficiently great compared to the capacitances of CO2 in blood and alveoli to permit rapid equilibration of CO2 across the alveolar–capillary membrane. As discussed (see below Carbon Dioxide) CO2 exchange requires a finite time for completion, but this delay is the result of the time needed to complete chemical and transport processes in blood, and is not the result of slow diffusive transport across the alveolar–capillary membrane.
Gases transported in blood only in dissolved form are exchanged almost instantaneously across the alveolar–capillary membrane. As long as gas solubilities in the membrane and blood are similar, diffusion equilibrium between alveolar contents and blood is reached within 0.01 second because the normal alveolar–capillary membrane is extremely thin (median thickness of 0.3 μm). Only gases such as oxygen and carbon monoxide that have large alveolar and blood capacitances and reduced solubility in the alveolar–capillary membrane will require a finite time to reach diffusive equilibrium.
CHEMICAL REACTIONS OF GASES
Transport of respiratory gases entails numerous chemical reactions with components of the blood. Like diffusive transport of oxygen, these chemical reactions are not instantaneous and require finite periods of time to reach completion. It is commonly thought that diffusion provides the greatest time-dependent impediment to gas exchange, but in actuality, chemical processes, especially those occurring in combination with diffusion or other chemical reactions, are more likely to slow rates of exchange.
OXYGEN AND CARBON MONOXIDE
From a stoichiometric viewpoint, the successive binding of O2 to the heme moieties of hemoglobin is described by successive steps, each with separate association and dissociation rate constants. If the heme rings acted independently, these constants would be the same for each heme ring and the resulting dissociation curve would have a hyperbolic shape. However, binding of oxygen to one of the heme rings affects the affinity for O2 of the remaining heme moieties of the molecule, leading to the familiar sigmoid shape of the oxygen dissociation curve.
Reactions of oxygen and hemoglobin during capillary transit are further complicated by the rate of oxygen diffusion through the viscous interior of the red cell. The chemical reactions of oxygen and hemoglobin occur quite rapidly in dilute hemoglobin solutions but proceed more slowly in red cell suspensions. Because of the large size of the hemoglobin molecule and increased viscosity of the red cell contents, hemoglobin remains relatively immobile. As the red cell enters the pulmonary capillary, oxygen molecules bind to reduced hemoglobin molecules just inside the erythrocyte membrane. As these hemoglobin molecules become saturated, subsequent oxygen molecules entering the red cell must diffuse more deeply into the interior of the cell to reach reduced hemoglobin molecules. This combination of diffusion and chemical reaction causes oxygen uptake to occur as an “advancing front” that proceeds at a rate that is an order of magnitude slower than O2 uptake in well-mixed, dilute hemoglobin solution. This combined process is complex and not easily described from a theoretical standpoint. As a result, the rate of oxygen uptake by hemoglobin contained in red cells is described by a single overall descriptive parameter, θO2, which incorporates all the processes into a single phenomenological value. θO2 varies with oxygen saturation, pH, and hemoglobin type. The same approach is used to describe carbon monoxide uptake in blood. The rate at which CO replaces bound O2 in blood with a normal hemoglobin concentration is described by θCO.
The rates of O2 and CO uptake by erythrocyte suspensions are determined in vitro and assumed to be representative of the rates of gas exchange in vivo. However, measurements of these rate constants in red cell suspensions in vitro may be affected adversely by methodological artifacts. The actual rates of combination of O2 and CO with red cells in vivo have not been measured and this lack of data leads to uncertainties in our understanding of exchange of the two gases in the lung.
CARBON DIOXIDE
CO2 is transported in blood as dissolved molecular CO2, bicarbonate ion, and carbamate ion.2 The latter is a salt of a carbamic acid formed by reaction of CO2 with terminal amino groups of the four chains comprising the hemoglobin molecule. The relation between the partial pressure of CO2 and the total content of CO2 in all forms is described by the CO2 dissociation curve of blood (see Chapter 15, Fig. 15-4). Because CO2 is more soluble than O2 in the alveolar–capillary membrane, it often is assumed that CO2 exchange occurs much more rapidly than O2 exchange. However, only dissolved CO2 can cross the alveolar–capillary membrane, and conversion of bicarbonate and carbamate to dissolved CO2 limits the rate of CO2 exchange. As indicated in Figure 16-1, Upper Panel, when a bolus of dissolved CO2 is injected into an isolated lung perfused with saline buffer, CO2 is rapidly exchanged similar to the inert gas acetylene.3 In contrast, when a bicarbonate bolus is injected into the same preparation (Fig. 16-1, Lower Panel), CO2 exchange lags behind acetylene excretion because a finite period of time is required to convert bicarbonate into dissolved CO2 that can cross the alveolar–capillary membrane.
Figure 16-1 Rates of acetylene (blue curves) and CO2 (red curves) excretion after two pairs of injections into the pulmonary artery of a single isolated lung preparation perfused with buffer. Volume changes are normalized to facilitate comparison. Upper Panel: The excretion of both acetylene and CO2 proceed at the same rate after injections of buffer containing either dissolved CO2 or acetylene. Differences in mean transit times for CO2 and acetylene were –0.027 second for first pair and +0.022 second for second pair of injections. Thus, CO2 crosses the alveolar–capillary membrane at the same rapid rate as the inert gas acetylene. Lower Panel: The excretion of CO2 generated from injections of bicarbonate (red curves) lags behind the excretion of dissolved acetylene (blue curves) by +0.301 second in the first set of paired injections of acetylene and bicarbonate and 0.312 second in the second set of paired injections. This slower excretion of CO2 is caused by the time required to convert bicarbonate to CO2 with catalysis in this experiment provided by carbonic anhydrase localized to the capillary endothelium. (Reproduced with permission from Schunemann HJ, Klocke RA: Influence of CO2 kinetics on pulmonary carbon dioxide exchange. J Appl Physiol. 1993;74:715.)
As blood enters the pulmonary capillary bed, dissolved CO2 immediately diffuses into the alveoli and capillary blood PCO2 falls to the level present in the alveolar gas. The majority (>85%) of the CO2 content in blood entering the capillary bed exists as bicarbonate ion.2,4 The rapid decrease in capillary PCO2 disturbs the equilibrium between bicarbonate ion and dissolved CO2 in both the plasma and the red cell. Bicarbonate ion combines with hydrogen ion extremely rapidly to form carbonic acid (H2CO3). The natural rate of dehydration of carbonic acid to CO2 and water is a slow process, requiring 60 to 90 seconds to reach completion. However, the reaction is catalyzed inside the red cell by a factor of ∼15,000 by the large concentration of carbonic anhydrase (CA) in red cells.
The substantial buffering capacity of hemoglobin inside the erythrocyte, augmented by the simultaneous conversion of reduced to oxygenated hemoglobin, provides the hydrogen ions required for this reaction.4 The dissolved CO2 formed by this reaction inside the red cell immediately leaves the blood and enters the alveoli.
There is a minimal amount of CA attached to the interior surface of the pulmonary capillaries, but its activity is <1% of the erythrocytic enzyme. As a result, little or no conversion of bicarbonate to dissolved CO2 occurs in the plasma during the short pulmonary capillary transit time (0.75 second at rest, 0.5 second during exercise). Inside the red cell, the catalyzed formation of dissolved CO2 rapidly (∼0.1 second) depletes the concentration of intracellular bicarbonate and the production of CO2 slows substantially1,5 However, as the intracellular concentration of bicarbonate decreases, plasma bicarbonate enters the cell in exchange for intracellular chloride. Bicarbonate–chloride movement across the erythrocyte membrane occurs in an electrically neutral, one-for-one exchange that is facilitated by an anion exchange protein present in the erythrocyte membrane.2 Despite the presence of approximately one million anion exchangers with an extremely rapid turnover (50,000 ions per second) in each red cell membrane, bicarbonate–chloride exchange requires 0.3 to 0.4 second to reach completion.1
Besides exchange of dissolved CO2 and bicarbonate, a modest amount of CO2 excretion (13%) results from release of CO2 bound to hemoglobin as carbamate.2 The release of CO2 bound as carbamate is caused by the alteration of the molecular conformation of hemoglobin that accompanies oxygenation. The carbamate reaction is complete in 0.2 to 0.3 second, but cannot occur until hemoglobin is first oxygenated. The process of oxygenation further delays conversion of carbamate to dissolved CO2.
Thus, exchange of CO2 in the lung is thought to require at least ∼0.4 to 0.5 second because of the series of processes that are necessary to convert CO2 carried as bicarbonate and carbamate to dissolved CO2.1,5 In fact, computational models of CO2 exchange suggest that in some circumstances exchange is not quite completed prior to blood leaving the pulmonary capillary.1,5 Even in the worst case, the degree of disequilibrium is small and a minimal increase in ventilation can easily compensate for a slight impairment of CO2 exchange.
SOURCES OF CO2 EXCRETED IN THE LUNGS
Bicarbonate accounts for the majority (>85%) of the total CO2 content transported in arterial and venous blood. Dissolved CO2 (∼5%–6%) and carbamate (<10%) contribute substantially less to the total CO2 content of blood. The quantity of bicarbonate transported in plasma is much greater than that carried within the red cells for three reasons. First, plasma volume constitutes 55% and erythrocytes only 45% of the total blood volume. Second, bicarbonate is present only in the volume of water in each component; plasma water content (95%) is substantially greater than erythrocyte water content (72%). Third, because of the Donnan distribution of anions across the erythrocyte membrane, bicarbonate concentration inside the red cell is only 63% of the plasma concentration.
The relative contributions of each form of CO2 content to the quantity of CO2 excreted during gas exchange in the lung are not exactly proportional to their blood contents. Analysis of the differences between arterial and venous blood CO2 contents can be used to calculate the amounts of each form of carbon dioxide content excreted in expired ventilation.2,6,7 This analysis of differences between arterial and venous blood CO2 contents is shown in Figure 16-2. The vast majority of expired CO2 enters the lung as bicarbonate and is converted into CO2 during capillary transit. The contribution of plasma bicarbonate (49%) is greater than the erythrocytic bicarbonate (30%) that is converted into CO2 and excreted in expired ventilation. Essentially all the CO2 derived from bicarbonate, whether in plasma or erythrocytes, has to be exposed to CA within the red cells to allow for reaction catalysis during the brief capillary transit. This emphasizes the importance of anion exchange across the erythrocyte membrane in the process of carbon dioxide excretion.
Figure 16-2 Sources of carbon dioxide excreted during passage of blood through the lungs in resting humans. The bars indicate the quantities of the different sources of CO2 leaving blood from red cells (red) and plasma (yellow). Bicarbonate contributes the overwhelming majority of CO2
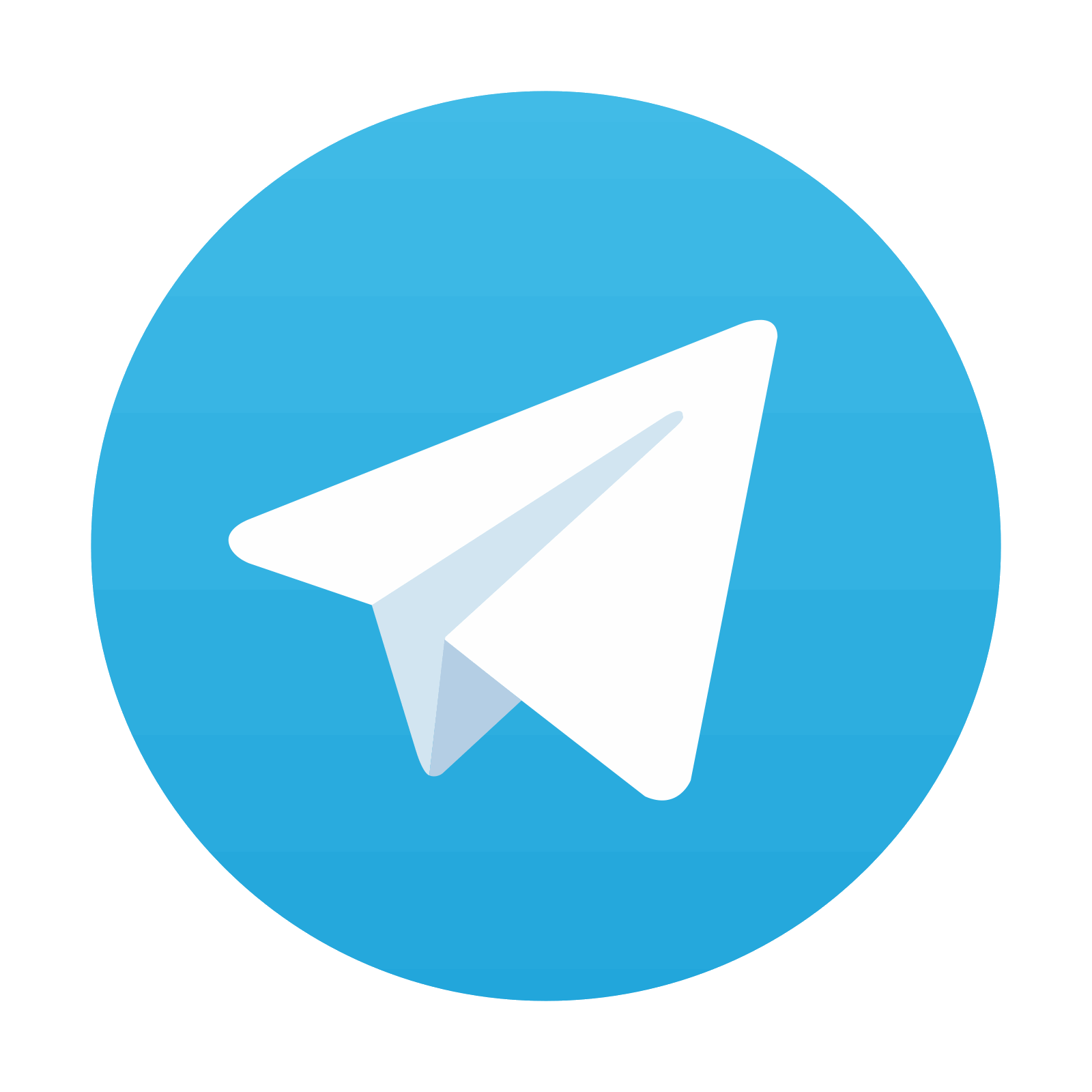
Stay updated, free articles. Join our Telegram channel
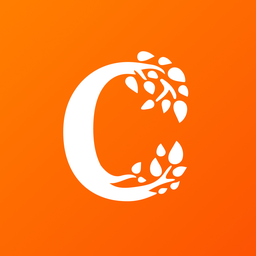
Full access? Get Clinical Tree
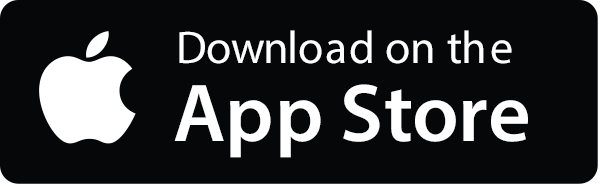
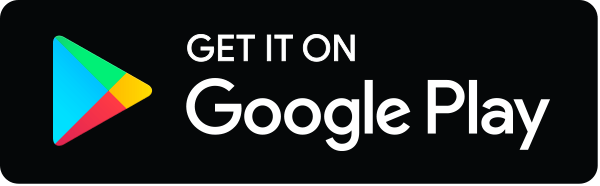