Physiology of Diastole
- Sherif F. Nagueh, MD
Left ventricular (LV) diastole begins with the closure of the aortic valve (AV), which ushers the drop in LV pressure. The time interval between AV closure and mitral valve opening is the isovolumetric relaxation time (IVRT). During that time, LV pressure is decreasing while its volume is unchanged (in patients without mitral and aortic regurgitation). This period ends with the opening of the mitral valve (MV). MV opening follows the drop in LV pressure below left atrial (LA) pressure. LV filling during the early diastolic filling period occurs as LV relaxation leads to lower LV early diastolic pressures and a positive transmitral pressure gradient. With ongoing LV filling, LA pressure drops and LV pressure rises, leading to a decreased transmitral pressure gradient and reduced LV filling. The rate of decline in early diastolic filling is related to LV stiffness such that higher LV stiffness leads to faster deceleration of LV filling. In late diastole, the LA contracts and leads to another positive transmitral pressure gradient and another peak of LV filling in late diastole ( Fig. 38.1 ).

LV relaxation
LV relaxation is affected by load, inactivation, and asynchrony. Increased LV afterload (LV end systolic wall stress) leads to delayed and slow relaxation. The effects of asynchrony have been examined in animal models as well as human disease, including patients with aortic stenosis, those with hypertension, and those with hypertrophic obstructive cardiomyopathy. There are data showing an improvement in LV relaxation with a reduction in LV dyssynchrony. It is also worth noting that increased load can affect LV relaxation both directly and indirectly because it can cause and aggravate dyssynchrony. Inactivation refers to the mechanisms leading to actin-myosin detachment and reduced calcium level in the sarcoplasm.
In ventricles with normal relaxation, LV minimal pressure is low, whereas with impaired relaxation this pressure is increased. Both the rate and the extent of LV relaxation affect LV diastolic pressures. The effect of impaired LV relaxation on LV filling pressures is more notable at fast heart rates. In this situation, LV filling is reduced (which can be detected by imaging) along with increased LV diastolic pressures. LV systolic duration is another important factor that affects filling pressures. For any given degree of LV relaxation, LV filling pressures increase as systolic duration increases.
LV relaxation is measured invasively by the time constant, tau (τ). The relation between LV pressure and time can be mathematically represented by several models. These include monoexponential decay to a zero asymptote, monoexponential decay to a nonzero asymptote, linear fit between LV pressure and its differential (dP/dt), and a hybrid logistic regression model. Another approach to assess LV relaxation includes the time for dP/dt to decline to 50% of its initial value (T 1/2 ). Of the methods just mentioned, the monoexponential decay of LV pressure to a zero asymptote has been most frequently used, and LV relaxation would be considered complete after 3.5 τ. The equation is given by: P (t) = P o e − t/τ , where P o is LV pressure at time of dP/dt min. Taking the natural log of both sides: ln P(t) = ln P o − t/τ, or t/τ= ln P o − ln P t . Therefore, τ can be derived as: t/(ln P o − ln P t ). At the time of mitral valve opening, t = IVRT, and τ can be given by: IVRT/(ln P o − ln P LAP ). It is possible to use noninvasive estimates of LA pressure (LAP) and LV end-systolic pressure and thus obtain t using entirely noninvasive measurements. This approach has been validated against invasive standards, although it has its limitations.
On the cellular level, there are several factors that affect relaxation. These include calcium transport into the sarcoplasmic reticulum (SERCA 2a), outside the cell (sodium calcium exchanger and calcium pump in the sarcolemma), and into the mitochondria; energy levels (ADP/ATP ratio and inorganic phosphate); the phosphorylation status of troponin I (this reaction desensitizes the contractile proteins to calcium); and myosin heavy chain mutations. The foregoing factors are affected by the sympathetic nervous system and circulating catecholamines, levels of atrial natriuretic peptide (ANP) and brain natriuretic peptide (BNP), the renin-angiotensin-aldosterone system, and inducible nitric oxide. In particular, active reuptake of calcium into the sarcoplasmic reticulum by SERCA 2a is reduced in patients with heart failure. The activity of SERCA 2a is under control by phospholamban, the phosphorylation of which releases the inhibitory effect of the protein on SERCA 2a activity.
LV stiffness
LV stiffness determines the diastolic volume pressure relationship. It is possible to derive LV operating chamber stiffness (dP/dV) using conductance catheters that simultaneously measure LV volume and pressure. Several factors affect chamber stiffness, including LV geometry, myocardial stiffness, and factors extrinsic to the LV such as pericardial, RV, and LV interactions. Of note, incomplete relaxation can contribute to elevated LV diastolic pressures for any given volume. Myocardial stiffness is determined by the sarcomeric proteins, microtubules, and extracellular matrix composition.
The diastolic pressure-volume relationship has been represented by several mathematical models including exponential, cubic, and power models. The exponential models have been more frequently used, as, for example: P = b e kV . Differentiating this equation: dP/dV = k (b e kV ) or dP/dV = k P, where k is the chamber stiffness constant. For any given volume, LV diastolic pressure is higher for stiffer ventricles. When data points are selected to derive k , end-diastolic volume and pressure data should be obtained, which necessitates altering LV preload. This can occur by varying venous return through inflating a balloon in the inferior vena cava. Some investigators used pressure and volume points from a single cardiac cycle. However, there are several problems with this approach. These include limited number of points, including the effects of relaxation as early diastolic data points are represented, and failing to detect dynamic changes in stiffness, as stiffness can be load dependent in a number of cardiac diseases.
Notwithstanding the foregoing methods that are used to compare stiffness between different ventricles, it is important to note that for any given ventricle, as LV volume increases, LV diastolic pressure rises steeply. This is due to the curvilinear relation between LV volumes and pressures. Therefore, for any given segment of a unique pressure-volume curve, one can express the PV relation by the operating chamber stiffness, which is lower in the flat portion of the curve and is higher in the steep segment at larger LV volumes ( Fig. 38.2 ).

On a cellular level, myocardial tension is primarily determined by titin (TTN). TTN has isoforms, the ratio of which determines passive tension and passive stiffness. Of note, TTN phosphorylation can modulate myocardial stiffness; this has been shown in animal models as well as in patients with heart failure. In the normal heart, collagen does not affect tension at the normal sarcomere length. However, higher levels of collagen isoform I and increased collagen cross-linking contribute to increased stiffness in patients with heart failure. Recent studies have reported abnormalities as well in matrix metalloproteinases with respect to their synthesis and degradation.
Ventricular arterial coupling
Increased arterial elastance (Ea) can contribute to the development of diastolic heart failure (DHF). Ea is derived as the ratio between end systolic pressure and LV stroke volume. In turn, end systolic pressure can be reliably estimated as: 0.9 × systolic blood pressure. Furthermore, the ratio of Ea to LV end systolic stiffness (Ees) can be derived and used to assess ventricular arterial coupling. With aging, both Ea and Ees increase. Some reports have noted abnormal Ea/Ees ratio with DHF, whereas others have not.
Diagnosis of diastolic heart failure
This diagnosis is established in the presence of symptoms and signs of heart failure, usually with an LV end-diastolic volume index less than 97 mL/m 2 and ejections fraction greater than 50%. Evidence of abnormal LV diastolic function is needed and can be established by invasive and noninvasive criteria (see following chapters). Invasive measurements include mean wedge pressure greater than 12 mm Hg, or LV end-diastolic pressure greater than 16 mm Hg, or τ greater than 48 msec, or LV chamber stiffness constant greater than 0.27. It is important to carefully establish this diagnosis, as it is not uncommon to have several other reasons for dyspnea in the elderly population, and a DHF diagnosis signifies high mortality and morbidity.
Methods of Assessment
- Monica Mukherjee, MD
- Theodore Abraham, MD
With the increasing number of patients with heart failure and preserved ejection fraction, and continued focus on reducing the rate of associated hospital admissions, comprehensive assessment of diastolic parameters is a particularly important aspect of cardiac testing. Diastole is known to occur in four distinct phases: isovolumetric relaxation, early diastolic filling, diastasis, and atrial filling. Active relaxation begins some time before the closure of the aortic valve, and in fact, as the left ventricle (LV) is unloading, it is already beginning to relax. Isovolumetric relaxation is defined as the decline in LV chamber pressure from the period of aortic valve closure prior to the opening of the mitral valve, without change in LV volume. The isovolumetric relaxation time (IVRT) encompasses the first 70 to 90 msec of diastole and is a marker of LV compliance. When LV pressure decreases below that in the left atrium (LA), isovolumetric relaxation ends and the mitral valve opens with negative suction, leading to rapid early filling of the LV and the mitral E-wave. As the pressure between the LA and LV equilibrates, mitral flow is interrupted, in a phase known as diastasis, which is dependent on a number of factors, including preload and the geometry, compliance, and stiffness of the LV chamber. Atrial systole represents the final phase of diastole, when the remaining volume within the LA is ejected into the LV, generating the mitral A-wave. Late diastolic filling is affected by LV compliance and LA contractile function. Following atrial contraction, LA relaxation begins, and the pressure within the LA begins to decrease below the level of the LV, causing the mitral valve to close.
Invasive assessment of diastolic function
Assessment of diastolic function can be performed invasively at the time of left cardiac catheterization using a high-fidelity micromanometer catheter placed in the LV. There are three parameters of diastolic function that can be reliably measured using invasive techniques. The first is isovolumetric relaxation, defined as the decline in LV chamber pressure from the period of aortic valve closure prior to the opening of the mitral valve. Isovolumetric relaxation, as with other measures of diastolic function, is highly dependent on loading conditions and may be further limited by underlying conditions such as hypertrophic cardiomyopathy, in which midsystolic obstruction interrupts pressure decay. The second parameter is end-diastolic pressure, which reflects the LV pressure at maximal LV volume immediately before opening of the aortic valve and the onset of ventricular contraction. Last, compliance or LV chamber stiffness can be measured and is derived from the relationship of volume to change in pressure over change in time (dP/dT).
Noninvasive assessment of diastolic function: echocardiography
Two-dimensional (2D) echocardiogram can be used in diastolic heart failure to assess LA and LV chamber size and function, LV hypertrophy, and Doppler assessment of diastolic function using transmitral and pulmonary vein velocities in conjunction with tissue Doppler imaging (TDI). The American Society of Echocardiography (ASE) 2009 guidelines recommend assessment of transmitral velocities by placing the pulsed wave (PW) Doppler cursor at the mitral valve leaflet tips in the apical four-chamber view, with an ideal sample volume of approximately 1 to 3 mm. Color Doppler can be used to aid with optimal alignment of the Doppler beam. In addition, continuous wave Doppler should also be used to ensure that the maximal transmitral velocities are being recorded. Increasing sweep speed to 100 mm/sec and adjusting the wall filter settings can further optimize Doppler signals.
Using the mitral inflow method, several diastolic parameters can be assessed, including early diastolic and late diastolic filling velocities, known as the E-wave and A-wave, respectively, as well as ratio of E to A, IVRT, and the deceleration time (DT) of the E-wave. The early diastolic E-wave velocity represents the gradient between the LA and the LV and is therefore highly affected by preload conditions and relaxation properties of the LV. The A-wave, alternatively, reflects this gradient in late diastole and is affected primarily by the compliance and contractility of the LV. The relationship of the E and A waves in conjunction with DT can thus be used to identify patterns of diastolic function ( Figs. 39.1 and 39.2 ).


TDI can be used to acquire velocities along the medial and lateral aspects of the mitral annulus to assess left ventricular end-diastolic pressure (LVEDP) when used with E-waves (E/e′), and to determine the effect of LV relaxation properties on mitral inflow E-wave velocities. Sample volumes are placed within 1 cm of the septal and lateral mitral annulus with baseline adjusted, and sweep speeds set around 50 to 100 mm/sec at end-expiration. Normal ranges of septal and lateral e′ velocities are less than 8 and less than 10, respectively ( Fig. 39.3 ). When both septal and lateral e′ velocities can be reliably obtained, it is important to take the average of both measurements. Because TDI requires tracking of the longitudinal motion of the mitral annulus toward the LV apex, E/e′ ratio has decreased accuracy in patients with mitral annular calcification, mitral stenosis, and/or prosthesis, and in cases of constrictive pericarditis.

Several ancillary measures can be used to distinguish between different grades of diastolic dysfunction. First, PW Doppler of pulmonary venous flow can be obtained by placing a sample volume approximately 0.5 cm within the right upper pulmonary vein and lowering the wall filter settings to allow for visualization of atrial reversal velocity waveforms. Normal pulmonary venous flow demonstrates systolic predominance (S-wave) or antegrade flow within the pulmonary veins during atrial diastole and ventricular systole ( Fig. 39.4 ). As mentioned earlier, early diastolic filling following the opening of the mitral valve generates the mitral inflow E-wave, which creates a D-wave or diastolic velocity within the pulmonary veins. In late diastole, atrial contraction generates the mitral inflow A-wave, which within the pulmonary veins appears as retrograde flow, or atrial reversal velocity (Ar). With progressive diastolic dysfunction, increasing LA pressure leads to increased reliance on atrial contraction to empty the residual volume within the LA. Within the pulmonary veins, this is manifested as increased D-wave velocity, or diastolic predominance. The duration of the Ar wave can also be subtracted from the duration of the mitral inflow A-wave; a result greater than 30 msec is an indicator of elevated LVEDP. Valsalva maneuver should also be performed during routine assessment of diastolic function. Valsalva decreases preload or LA pressure, and in a pseudonormal pattern, patients with diastolic dysfunction will revert to a grade I pattern with this maneuver. In patients with grade III restrictive filling pattern, decreased preload with Valsalva will result in a grade I impaired relaxation or may remain fixed, in what is known as a grade IV or fixed restrictive pattern ( Fig. 39.5 ).


Color M-mode flow propagation velocity is another method of assessing diastolic function using echocardiography ( Fig. 39.6 ). In the apical four-chamber view, the M-mode is placed in long axis through the mitral inflow tract and LV apex, with the cursor placed approximately 4 cm within the LV chamber. The Nyquist limit is then set such that the highest velocity along the central jet appears blue. Flow propagation velocity ( V p ) is defined as the slope of the first aliasing velocity during early LV filling and is considered normal when V p is 0.5 m/sec or greater. This is known as the slope method, which appears to be the least variable of the methods available. Flow propagation velocities relate inversely to the time constant of LV relaxation, known as tau; however, this seems to be a relatively insensitive measure of load. The ratio of the mitral inflow E-wave to V p provides a semiquantitative estimate of mean LAP. E/ V p of 2.5 or greater correlates with an LVEDP greater than 15 mm Hg.

Of the numerous echocardiographic parameters mentioned, the ASE guidelines recommend that mitral inflow velocity ratios of E/A, e′ as an estimation of LV filling pressure, and deceleration time are the highest value in the assessment of the presence and grade of diastolic dysfunction.
Echo Doppler Parameters of Diastolic Function
- Teerapat Yingchoncharoen, MD
- Chanwit Wuttichaipradit, MD
- Allan L. Klein, MD
- Chanwit Wuttichaipradit, MD
Diastolic heart failure, or heart failure with preserved ejection fraction (HFpEF), is not only a commonly encountered syndrome that accounts for approximately 50% of all heart failure, but a well-recognized contributor to clinical heart failure with increased morbidity and mortality similar to systolic heart failure. Diastole is a complex and dynamic phenomenon that is influenced by age, loading condition, heart rate, and peripheral vascular tone. Routine assessment of diastolic function as a part of comprehensive echocardiography was recommended by the American Society of Echocardiography (ASE) and the European Association of Cardiovascular Imaging guidelines. The purpose of this chapter is to describe the Doppler parameters of diastolic function used in clinical practice and their prognostic implications.
Doppler mitral flow velocity patterns
Doppler measurement of the mitral flow velocity provides unique information about the velocity of blood flow across the mitral valve into the ventricle. This velocity is a complex function of the pressure gradient across the mitral valve, described in the law of conservation of energy equation. Hence, flow velocity represents the intermediate link between hemodynamic conditions indicated by instantaneous left atrial and left ventricular pressures and the filling characteristics of the ventricle. Mitral flow velocity variables are recorded from the apical four-chamber view with pulsed wave (PW) Doppler by placing a 1- to 2-mm sample volume between the mitral leaflet tips at their narrowest point, which is visualized with two-dimensional echocardiography (2DE) at end expiration during normal breathing. The Doppler gain and filter settings should be as low as possible, with sweep speed at 50 to 100 mm/sec and the spectral Doppler baseline one third to halfway up on the monitor display. Variables that should be measured include peak mitral flow velocity in early diastole (E wave) and during atrial contraction (A wave), mitral deceleration time (DT), the E wave velocity just before atrial contraction (E at A), the duration of mitral A wave velocity (Adur) (sample volume at the mitral annulus level), and isovolumetric relaxation time (IVRT).
In young, healthy individuals, there is a rapid acceleration of blood flow from the left atrium (LA) to the left ventricle (LV). Early peak filling velocity of 0.6 to 0.8 m/sec occurs 90 to 110 msec after the onset of mitral valve opening. This E wave occurs simultaneously with the maximum pressure gradient between the LA and LV that in turn depends on the pressure difference along the flow stream, LV relaxation, and the relative compliance of the two chambers. Normal E wave pattern shows rapid acceleration and deceleration; normal deceleration slope is 4.3 to 6.7 m/sec 2 . Mitral DT, as defined by the time interval from the peak E wave to its extrapolation to baseline, typically ranges from 150 to 240 msec. DT is prolonged in patients with LV relaxation abnormalities because it takes longer for LA and LV pressure to equilibrate. A low normal DT, on the other hand, can be seen in normal young subjects, in whom there is vigorous LV relaxation and elastic recoil, and a short DT if there is a decrease in LV compliance or marked increase in LA pressure as in advanced diastolic dysfunction (DT less than 150 msec). Early diastolic filling is then followed by a variable period of minimal flow (diastasis). The duration of diastasis is dependent on heart rate; it is longer with slow heart rates and entirely absent with faster rates. Lastly, the A wave, which is the result of an atrial kick pushing the remaining blood from the LA to the LV, follows the diastasis and is influenced by LV compliance and LA contractility ( Fig. 40.1 ). The normal A wave velocity typically ranges from 0.19 to 0.35 m/sec and is significantly smaller than the E wave, resulting in an E/A ratio greater than 1. Sinus tachycardia, premature atrial contraction, and first-degree atrioventricular block may result in fusion of the E and A waves. The peak A wave velocity in fused E and A velocity, with an E-at-A wave velocity greater than 20 cm/sec is larger than it would have been at a slower heart rate, when mitral flow velocity has time to decrease before atrial contraction. In these cases, the E/A wave ratio may be reduced, compared with values obtained at a slower heart rate, so that more reliance on other Doppler variables is needed when interpreting the fused LV filling pattern.

With aging, the LV relaxation takes longer, primarily because there is a gradual increase in systolic blood pressure and LV mass, resulting in reduced LV filling in early diastole and increased filling at atrial contraction. The peak E and A wave velocities become approximately equal during the sixth and seventh decade of life. DT and IVRT become longer with age, and atrial contraction contributes up to 35% to 40% (as opposed to 10% to 15% in adolescents) of LV diastolic stroke volume.
With progressively worsening diastolic function, transmitral flow evolves in a recognizable pattern. In grade 1 diastolic dysfunction (abnormal relaxation), there is a low E wave and a high A wave, resulting in an E/A ratio less than 1. DT is prolonged and is usually greater than 240 msec, and IVRT (measured by pulsed or continuous wave Doppler) is longer than 90 msec. Grade 2 diastolic dysfunction, or pseudonormal pattern, is associated with a normal appearance of the transmitral inflow with an E/A ratio between 1 and 1.5, a DT between 150 and 200 msec, and an IVRT greater than 90 msec. With disease progression (grade 3 diastolic dysfunction or restrictive filling), there is a very high E wave, a low A wave, and a significantly decreased DT. The E/A ratio is usually greater than 2, DT is less than 150 msec, and IVRT is longer than 70 msec. Further observations have subcategorized this last pattern to either reversible or fixed restrictive pattern (grade 4) depending on the response to the Valsalva maneuver or other preload reducing maneuvers. Doppler criteria used to define grades of diastolic dysfunction are summarized in Table 40.1 .
Criteria | Normal Young | Normal Adult | Impaired Relaxation (Grade 1) | Pseudonormal (Grade 2) | Reversible Restrictive (Grade 3) | Irreversible Restrictive (Grade 4) |
---|---|---|---|---|---|---|
E/A ratio | 1-2 | 1-2 | < 1.0 | 1-1.5 (reverses with Valsalva maneuver) | > 1.5 | 1.5-2.0 (Doppler values similar to grade 3 except no change with Valsalva maneuver) |
Deceleration time (msec) | < 240 | 150-240 | ≥ 240 | 150-200 | < 150 | < 150 |
IVRT (msec) | 70-90 | 70-90 | > 90 | < 90 | < 70 | < 70 |
PV S/D ratio | < 1 | ≥ 1 | ≥ 1 | < 1 | < 1 | < 1 |
PV AR-MV A wave duration (msec) | ≥ 30 | ≤ 0 | ≤ 0 or ≥ 30 | ≥ 30 | ≥ 30 | ≥ 30 |
AR velocity (cm/sec) | < 35 | < 35 | < 35 | ≥ 35 | ≥ 35 | ≥ 35 |
Propagation velocity (cm/sec) | > 55 | > 55 | > 45 | < 45 | < 45 | < 45 |
Mitral E′ velocity (cm/sec) | > 10 | > 8 | < 8 | < 8 | < 8 | < 8 |
The Doppler imaging of mitral inflow may have additional forward flow during mid-diastole. The prominent mid-diastolic filling “hump,” which has been described as a mitral L wave, which can be seen in healthy individuals with bradycardia, or it may be a pathologic change in patients with advanced diastolic dysfunction with elevated LV filling pressures.
Valsalva maneuver
Because diastolic function is affected by preload change, the Valsalva maneuver is a test used to modify cardiac loading condition, which is helpful in the measurement of mitral inflow parameters. The Valsalva maneuver is performed by forceful attempted expiration (about 40 mm Hg) against a closed mouth and nose, resulting in a complex hemodynamic process involving four phases. During the strain phase of the maneuver, preload (mean LA pressure) is reduced, and peak mitral E wave velocity decreases by at least 20% during maximum strain; there is also a smaller decrease in peak A wave velocity. With pseudonormal mitral flow patterns, the Valsalva strain lowers the elevated LA pressure and reveals the underlying impaired LV relaxation, resulting in a measured E/A less than 1. Patients with restrictive filling patterns or individuals who have a sensitivity to preload will revert to a pseudonormal or even impaired relaxation pattern. Patients who have restrictive filling patterns and exhibit no change with Valsalva have severe irreversible or fixed diastolic function. The primary limitation of routine use of Valsalva maneuver is that it is difficult to obtain adequate Doppler signal for measurement. In a previous report, satisfactory Doppler data could be obtained during Valsalva maneuver in only 61% of patients. Occasionally the position of the sample volume may move during the maneuver. In addition, the inherent difficulties in performing an adequate Valsalva maneuver may limit its use in routine practice.
Pulmonary venous flow
Accurate pulmonary vein (PV) flow velocity can be obtained from the apical four-chamber view with PW Doppler in 85% to 90% of patients. The right upper PV is the most frequently visualized and accessible from the transthoracic echocardiographic examination. To properly obtain the pulmonary vein flow with Doppler, the sample volume should be placed approximately 1 to 2 cm into the pulmonary vein, with box size adjusted to 3 to 4 mm, Doppler filter set to 200 Hz, and sweep speed adjusted to 50 to 100 mm/sec.
The flow from the PV to the RA occurs in three phases: antegrade systolic, antegrade diastolic, and retrograde following atrial contraction. The normal pulmonary venous waveforms are triphasic or quadriphasic. In 70% of patients, it is difficult to discriminate between the two systolic components of the PV flow. However, in patients with low filling pressures, systolic forward flow becomes biphasic, and the PV flow pattern is quadriphasic. The first phase is early systolic (PVs1). It occurs in early systole and represents the increase in pulmonary venous flow secondary to atrial relaxation. The second phase, late systolic (PVs2), occurs in mid to late systole. It is caused by the increase in pulmonary venous pressure propagated through the pulmonary arterial tree from the right side of the heart. The apical systolic annular motion of the mitral annulus is also believed to contribute to this finding. This phase reflects the reservoir function of the LA. The next phase is early diastole (PVd), which occurs during ventricular relaxation and is influenced by LV filling. It corresponds to transmitral E velocity and represents LA conduit function. The last phase is peak reverse flow velocity at atrial contraction (PVa or PV AR): it occurs in late diastole; is influenced by late diastolic pressures in the LV, atrial preload, and LA contractility ; and reflects the LA booster function ( Fig. 40.2 ).The PVa velocity and duration depend on atrial preload and contractility.

Systolic/Diastolic ratio
In normal adults, the PVs2 (S) wave should be greater than the PVd (D) wave and S/D ratio is greater than 1. However, the PV flow pattern is affected by several factors. In young adults (age younger than 40 years), the diastolic wave typically predominates, reflecting rapid diastolic suction and filling during myocardial relaxation. With increasing age, the S/D ratio increases. As LA pressure increases, particularly with elevated LV filling pressures, the S wave velocity decreases and the S/D ratio falls to less than 1. The PV systolic fraction, which is the ratio of the velocity-time integral (VTI) of the S wave to the sum of the S and D waves (PV systolic fraction = S VTI /[S VTI + D VTI ]), was shown to be the best predictor of LA pressure. A PV systolic fraction of less than 55% predicted a pulmonary capillary wedge pressure (PCWP) of 15 mm Hg or less, with a sensitivity of 91% and specificity of 87%. A reduced PV systolic fraction less than 40% is related to decreased LA compliance and increased mean LA pressure in the patients with poor LV systolic function. There is limited accuracy in patients with preserved LV systolic function (velocity greater than 50%), atrial fibrillation, significant mitral regurgitation, and hypertrophic cardiomyopathy.
Reverse Flow at Atrial Contraction Velocity
In normal subjects, reverse flow at atrial contraction (Ar) velocities increase with age but usually do not exceed 35 cm/sec. The increased Ar velocities greater than 35 cm/sec suggest elevated left ventricular end diastolic pressure (LVEDP). The prolonged time difference between Ar and mitral A wave duration (Ar-A) also indicates elevated LVEDP and can separate patients with abnormal LV relaxation into those with normal filling pressures and those with elevated LVEDPs but normal LA pressures. An Ar-A duration longer than 30 msec is predictive of an LVEDP greater than 20 mm Hg with high sensitivity (82%) and specificity (92%). Additionally, Ar-A duration is the only indication of increased LV A wave pressure that is independent of age and reliable in patients with depressed and preserved LV ejection fraction. Atrial fibrillation results in a blunted S wave and the absence of Ar velocity.
The primary limitation in interpreting PV flow is the difficulty in obtaining adequate Doppler signal, especially when assessing the Ar wave, which is often obscured by low-velocity LA wall motion.
Color M-mode flow propagation velocity
During isovolumetric relaxation, the LV pressure drops without LV filling. The time constant of LV pressure decline known as tau (τ) is an index of LV relaxation that is relatively independent of heart rate and preload. It is well recognized that in early diastole, small but significant intraventricular mitral-to-apex pressure gradients are generated; these gradients are proposed to exert a ventricular suction effect. Color M-mode velocity propagation (Vp) index, a noninvasive index of fluid acceleration, can mirror these intraventricular pressure gradients (IVPGs). It is also inversely related to the ventricular relaxation time constant τ: a faster rate of ventricle relaxation leads to a more rapid propagation of flow into the ventricle. Color M-mode Doppler is a pulsed Doppler technique in which mean velocities are color coded and exhibited in time (on the horizontal axis) and depth (on the vertical axis), providing a spatiotemporal map of flow velocity along the scan line. Acquisition is made at end expiration in the apical four-chamber view, using color flow imaging with a narrow color sector, and the depth is adjusted to include the entire LV from the mitral leaflets to the apex. The M-mode scan line is located through the midline of the LV inflow blood column from the mitral valve to the apex. Then the color flow baseline is adapted to lower the Nyquist limit to plus or minus 75% of the spectral E velocity to obtain overflow (“aliasing”) so that the central highest velocity jet is blue. The sweep speed of M-mode is 100 mm/sec. Vp should be measured as the slope of the first aliasing velocity (red to blue) during early filling, from the mitral valve plane to 4 cm distally into the LV cavity. A Vp less than 50 cm/sec is consistent with diastolic dysfunction. The most widely used index is E/Vp, which has been validated in the healthy population, and in people with hypertrophic cardiomyopathy, dilated cardiomyopathy, and ischemic heart disease. E/Vp greater than 1.5 can predict LVEDP greater than 12 mm Hg with high accuracy (sensitivity, 79%; specificity, 89%; positive predictive value [PPV], 93%; negative predictive value [NPV], 70%). In addition, the E/Vp ratio has also been applied in people with atrial fibrillation, and it is reasonably accurate for assessing LVEDP. The measurement should be averaged for more than three heartbeats, and an E/Vp ratio of at least 1.4 yields a 100% specificity and 72% sensitivity in predicting a PCWP greater than 15 mm Hg. There are several potential limitations of the use of Vp as a measure of LV diastolic function. First, in many situations, the isovelocity contour may not be accurately described by a straight line, and Vp varies with the method used to determine the isovelocity contour. Also, E/Vp should be interpreted with caution in patients with preserved LVEF, especially in patients with normal LV volumes, because abnormal filling pressures can have a misleading normal Vp. Additionally, there are reports showing a positive influence of preload on Vp in patients with normal LVEF as well as those with depressed LVEF.
Tissue doppler annular velocity
The velocity of the mitral annulus represents velocity of changes in the LV long-axis dimension. In systole the mitral annulus moves toward the LV apex. In diastole it returns to its initial position in two waves, rapid filling and atrial contraction. This manifests on the tissue Doppler signal of mitral annulus as s′ (systolic velocity), e′ (early diastolic velocity), and a′ (late diastolic velocity). The measurement of mitral annular velocities is an important component in interpreting the diastolic filling pattern, estimating LV filling pressures, and differentiating constrictive pericarditis from restrictive cardiomyopathies. These velocities are recorded from the apical four-chamber view by placing a 5- to 6-mm sample volume over the lateral or medial portion of the mitral annulus to cover the longitudinal excursion of the mitral annulus in both systole and diastole. The velocity scale should be set at about 20 cm/sec above and below the zero-velocity baseline, and the angulation between the ultrasound beam and the plane of cardiac motion should be minimal. The recommendation for spectral recordings is a sweep speed of 50 to 100 mm/sec at end expiration, and measurements should be averaged for at least three consecutive cardiac cycles.
The velocity of mitral annulus movement during early filling (e′) correlates modestly with the invasively measured time constant τ, but it is not solely determined by myocardial relaxation. The e′ velocity is a measure of myocardial relaxation that is relatively independent of preload in patients with cardiac disease, whereas it appears load-dependent in patients with normal systolic function. In healthy and young persons, septal e′ is greater than 10 cm/sec and lateral e′ is greater than 12 cm/sec. These velocities increase with exercise and underlie diastolic LV suction. The e′ velocities fall with corresponding elevated E/e′ ratio because myocardial relaxation worsens with aging. In patients with diastolic dysfunction, e′ is even more reduced than with age and remains reduced in all grades of diastolic dysfunction. Typically the septal e′ is lower than the lateral e′ velocity. Septal E/e′ ratio of 8 or less is associated with normal PCWP, and an E/e′ ratio of 15 or higher suggests elevated PCWP. When the value is between 8 and 15, other echocardiographic indices should be used. Currently, if the lateral E/e′ ratio is used, E/e′ ratio of at least 12 is a marker of elevated LV filling pressures in patients with preserved LVEF. The lateral E/e′ may provide a more reliable estimate of elevated filling pressure in patients with preserved LVEF. The ASE guidelines for assessment of diastolic function recommend using an average of the septal and lateral annular velocities, where an average E/e′ ratio of 13 or greater indicates elevated filling pressures. However, there are certain situations where E/e′ may not provide an accurate assessment of PCWP, such as in the normal heart, where e′ behaves as a load-dependent variable; in constrictive pericarditis, where E/e′ does not increase despite elevated PCWP (annulus paradoxus) ; in mitral valve disease (mitral annular calcification, mitral stenosis, significant mitral regurgitation); and after mitral valve surgery (mitral valve annuloplasty or mitral valve replacement). Additionally, several studies with conflicting results have questioned the robustness of the E/e′ ratio in estimating filling pressures in the setting of acutely decompensated advanced systolic heart failure, especially in patients with dilated LV, severely impaired cardiac output, cardiac resynchronization therapy, and symptomatic hypertrophic cardiomyopathy.
Several studies have assessed the time interval between the onset of the mitral E velocity and annular e′ velocity as an alternative for assessing LV filling pressure. The ratio of isovolumetric relaxation time to the time interval between E and e′ velocities has been used to estimate filling pressures in patients with primary mitral valve disease. For prognostication, a decreased e′/a′ ratio (which is adjusted for systolic function by dividing the ratio by s′) is an independent predictor of mortality in the general population.
Myocardial performance index
Myocardial performance index, or Tei index, is based on Doppler-derived time intervals that combines both systolic and diastolic cardiac performance. Tei and colleagues have shown that the Tei index is simply derived using conventional pulsed wave Doppler or tissue Doppler echocardiography, as shown in ( Fig. 40.3 ). Interval a between cessation and re-onset of mitral filling flow includes isovolumetric contraction time (IVCT), ejection time (ET), and IVRT. Interval b between onset and cessation of aortic ejection flow equals ET. The mean normal value of the LV Tei index is 0.39 ± 0.05. In adults, values of the LV Tei index less than 0.40 are considered normal. Higher index values are correlated with more pathologic states of overall cardiac dysfunction. In systolic dysfunction, prolonged IVCT, prolonged IVRT, and a shortened ET were observed. In diastolic dysfunction, IVRT is the main feature.

The advantages of the Tei index are that it is noninvasive, easy to obtain, and reproducible. Moreover, a number of studies have proven that the Tei index is independent of arterial pressure, heart rate, atrioventricular valve regurgitation, ventricular geometry, afterload, and preload in patients who are supine. The Tei index has been shown to have robust prognostic value in several cardiac diseases, including dilated cardiomyopathy, pulmonary hypertension, cardiac amyloidosis, and myocardial infarction.
Similar to the other methods, the limitations of this technique include (1) the impracticality of assessing the Tei index in patients with atrial fibrillation, frequent supraventricular and ventricular extra systoles and intraventricular conduction disturbances, ventricular pacing, and significant atrial tachycardia with mixing of the two transmitral flow waves; (2) its partial dependence on preload, although it’s believed to be less dependent than other diastolic Doppler parameters; and (3) pseudonormalization of the index in patients who have restrictive physiology with preserved LV systolic function.
Integration of doppler echocardiography parameters
The diastolic filling pattern is separated into distinct categories and simplified into LV diastolic function grades, as outlined in Figure 40.4 , and take into consideration the effect of loading conditions in improving or worsening the diastolic filling pattern.

Conclusion
Integration of multiple Doppler echocardiography variables allow a comprehensive assessment of diastolic function in a wide variety of patients. It enables the diagnosis of underlying cardiac dysfunction, gives an estimate of LV filling pressures, guides heart failure therapy, and provides important prognostic information. The development of novel echocardiography techniques allow more advanced assessment of diastolic function; however, standard Doppler echocardiography remains the keystone of diastolic function assessment.
Estimation of Left Ventricular Filling Pressures
- Miguel A. Quinones, MD
The evaluation of left ventricular (LV) diastolic function has become an integral part of a routine echocardiographic study and complements the assessment of systolic function. As part of the evaluation of diastole, a statement regarding resting filling pressures should be included whenever possible to assist clinicians in evaluating patients with presenting symptoms of dyspnea or with known heart failure, coronary artery disease, or other forms of structural heart disease. In this chapter we present a practical algorithm based on concepts presented in the preceding three chapters and in “Recommendation for the Evaluation of Last Ventricular Diastolic Function by Echocardiography” by the American Society of Echocardiography. The algorithm allows detection of normal and elevated filling pressures with a good amount of certainty. It should be noted, however, that precise derivation of filling pressures by published equations is fraught with large margins of error, and in most cases, it is preferable to indicate if filling pressures are normal (mean left atrial pressure [LAP] is 12 mm Hg or less), mild to moderately elevated, or severely elevated.
The rise in LV pressure during diastole results from an interaction between active LV relaxation, inflow volumes, and chamber stiffness. An abnormal rise during the early rapid filling phase of diastole ( Fig. 41.1 ) is always associated with increases in left atrial (LA) and LV end-diastolic pressures (LVEDP), and it results from abnormal LV relaxation (in extreme cases, relaxation is not completed by end diastole) and increased stiffness. However, a rise in LVEDP may also occur in the presence of normal LAP as a result of increased late diastolic stiffness and a vigorous atrial contraction ( Fig. 41.2 ). Cardiac dyspnea is always associated with increased LAP; thus it is fortunate that most of the echocardiographic parameters discussed later are better at predicting LAP than LVEDP.

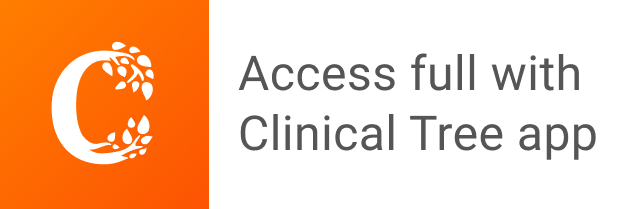