Development of the Heart: Morphogenesis, Growth, and Molecular Regulation of Differentiation
Aleksander Sizarov
H. Scott Baldwin
Deepak Srivastava
Antoon F.M. Moorman
Introduction
As the organ most essential for life, the heart is the first organ to form, and must function to support the rapidly growing embryo before it has the opportunity to shape itself into a four-chambered organ. Our ability to understand the mechanisms underlying the development of the heart has tremendously advanced, largely due to a plethora of new experimental studies. Although new editions of human embryology textbooks amend recent molecular insights (1,2,3,4), the description of cardiac morphogenesis is, unfortunately not updated, and has become increasingly schematic and alienated from the originally illustrated substrate. Consequently, many relevant details have diverged and have been lost. Nearly identical illustrations in chapters on cardiac development in virtually every modern human embryology textbook are reproductions of the original drawings of the developing human heart, published in the first half of the previous century (5,6,7,8,9,10,11,12) and simplified by Frank Netter (13). The advent of new methods used in experimental embryology, such as transgenic technology and molecular lineage tracing, has generated a wealth of new data on genetic and molecular determinants of cardiac development. The corollary was a growing lack of interest for the morphogenetic events themselves (14). In this chapter we present an up-to-date framework of cardiac morphogenesis, which incorporates important anatomic detail with current insights into the molecular regulatory pathways underlying cardiac development. The development of the cardiac pacemaking and conduction system, together with its functional maturation is described in detail in Chapter 18 within the Electrophysiology section of this Textbook.
From Cardiac Embryology to Understanding the Development of Congenital Heart Defects
Congenital malformations of the heart and great vessels contribute significantly to pediatric morbidity and mortality worldwide, particularly in the developed world (15,16,17,18). The wide spectrum of congenital cardiac defects occurring mostly in isolation, but also in various combinations, indicates the complexity of the regulation of cardiac morphogenesis (Fig. 1.1). The combination of complex morphogenetic and hemodynamic factors may contribute to the extreme sensitivity of the developing heart to perturbations in development. This phenomenon is reflected in the estimated 10% incidence of severe cardiac malformations observed in spontaneously aborted fetuses. Up to the recent past congenital malformations of the heart usually were classified according to embryologic concepts. It became an undisputable dogma that understanding cardiac morphogenesis would be an imperative for comprehensive competence in management of congenital heart defects. The inherent limitations of the past embryologic theories and the advent of palliative and corrective procedures led to a descriptive nomenclature of congenital heart defects, based on their anatomic and physiologic features governing therapy (19,20,21). Advances in molecular biology, genetics, and their application in lineage studies of the developing heart, however, have led to a renaissance of cardiac embryology and its significance for the understanding of congenital heart defects. The ability to go beyond anatomic descriptions of cardiac defects to the discovery of gene regulatory networks responsible for cardiac morphogenesis has opened vistas on future pediatric cardiology that will involve more directed therapeutic and preventive measures. Discrepancies in severity of cardiac malformations as seen in mouse models compared to human patients with mutations in the same genes remain, however, a serious problem for direct extrapolation of experimental findings into clinical setting. This might be related to the redundancy of the genetic regulation of heart development in human.
From Cardiac Embryology to Stem Cell Approaches to Regenerate the Failing Heart
The notion that genes involved in early heart formation may be redeployed to help protect, repair, or regenerate cardiac muscle,
has become a major driving force in efforts to understand early developmental pathways (22). The advent of stem cell technology along with the notion that stem cells might be an ideal source to repair the failing myocardium boosted the development of different techniques to study the genetic and molecular regulation of the formation of the primitive myocardial heart tube and its further differentiation. Deciphering nature’s secrets of heart formation might lead to novel approaches to repair or regenerate damaged heart muscle. Recent evidence has begun to support this idea and has led to increased interest in the early events of cardiac cell fate decisions and cardiomyocyte differentiation, migration, and survival. The potential of stem cells in regenerative medicine seems enormous, and insights into the natural process of cardiogenesis from progenitor cells during embryogenesis are thought to form the basis of reprogramming cells for therapeutic use (Fig. 1.2) (23).
has become a major driving force in efforts to understand early developmental pathways (22). The advent of stem cell technology along with the notion that stem cells might be an ideal source to repair the failing myocardium boosted the development of different techniques to study the genetic and molecular regulation of the formation of the primitive myocardial heart tube and its further differentiation. Deciphering nature’s secrets of heart formation might lead to novel approaches to repair or regenerate damaged heart muscle. Recent evidence has begun to support this idea and has led to increased interest in the early events of cardiac cell fate decisions and cardiomyocyte differentiation, migration, and survival. The potential of stem cells in regenerative medicine seems enormous, and insights into the natural process of cardiogenesis from progenitor cells during embryogenesis are thought to form the basis of reprogramming cells for therapeutic use (Fig. 1.2) (23).
Reports on the existence of niches of small, noncardiomyocyte populations in the postnatal heart, which upon isolation in culture can differentiate into cardiac muscle and endothelial cells, initially generated considerable excitement that the heart, like other organs, may have a resident pool of progenitor cells (24,25). However, isolation and characterization of these cells has proved elusive and the clinical utility has remained minimal. Perhaps the most exciting observation was from a series of experiments that demonstrated that skin fibroblasts from mice and humans could be reprogrammed or “induced” to form pluripotent stem cells (iPS) upon introduction of transcription factors that are known to regulate pluripotency of embryonic stem cells (26,27). Recently, it has been demonstrated that a mix of “cardiac” transcription factors is able to directly change the molecular and functional phenotype of adult fibroblasts into the primitive cardiomyocyte-like phenotype, rather than first generating a pluripotent intermediate (28,29). The ability to derive iPS cells from patients that can be differentiated into cardiomyocytes provided exciting potentials for disease-specific modeling and therapeutic pharmacologic screening. As yet, however, it has proved impossible to generate “mature” cardiomyocytes in contractile and electrophysiologic terms that also functionally are integrated within the syncytium of the host’s ventricular myocardium without becoming arrhythmogenic. However, continuing experimental work may realize the possibility of transforming cardiac fibroblasts into functionally integrated and mature cardiomyocytes in situ, providing thus a more immediate therapy for injured myocardium.
Experimental Animal Models and Tools Used in Cardiac Embryology Research
Scarcity of well-preserved human embryos necessitates the use of animal embryos to study the developing heart. Furthermore, detailed molecular analysis of cardiac development in humans is extremely difficult and experimentation on the human embryo is not possible. The recognition that cardiac genetic pathways are highly conserved across vastly diverse species from flies to man has resulted in an explosion of information from studies in more tractable and accessible biologic models. The most commonly used animal models to study cardiac development and developmental genetics include fruit fly, zebrafish, frog, chicken, and mouse models (Fig. 1.3). The fruit fly (Drosophila melanogaster) has become an important experimental model, leading to several important discoveries on the genetic regulation of the formation of the myocardium. Drosophila has rapid breeding times; it has a simple genome with usually just a single set of genes that often have three or more homologs in vertebrates. Its DNA can be chemically mutated in a random fashion, allowing subsequent phenotypic analysis and reverse genetics, which results in the identification of mutations associated with distinct developmental defects. Because of obvious limitations posed by the very simple organization of the fly’s “heart,” other experimental model systems are required, allowing similar chemical mutagenesis efforts in the context of more complex cardiac morphology. Zebrafish (Danio rerio) and frog (Xenopus laevis) are vertebrate species having a two- and three-chambered heart, respectively, which is easily visible and is not necessary for survival of embryo during the early periods of cardiac development, clearly posing important advantages for experimental studies.
The anatomic organization of the fruit fly, zebrafish, and frog is, nonetheless, different from human embryos to such an extent that direct comparison of developmental stages is not possible. Morphogenetic events during cardiac development in two other widely used experimental animal models, chicken and mouse, are quite similar to those in human, which allow assessment of comparable stages in these species (30,31,32,33) (Table 1.1). Although genetic approaches are not feasible in the chick, the embryos are easily accessible within the egg for surgical and molecular manipulation during cardiogenesis, which has been very useful in cell fate analyses and defining the role of populations of cells during development. Finally, use of the laboratory mouse, a mammal with a cardiovascular system highly comparable to that of the human, has been invaluable in understanding the mechanisms underlying formation of the heart and its structures by the molecular manipulation of specific genes. Use of transgenic mouse models particularly has allowed identification of the genes essential for mammalian cardiac development. Thus, each experimental animal offers unique opportunities to deepen our understanding of cardiogenesis. Although the ease of genetic manipulation posed by the experimental animal models has been and continues to be an enormous help in studying heart development, one should also realize the intrinsic limitations of these hearts, which are not identical to the human heart (Fig. 1.3).
Different tools can be used to analyze the developing heart. Historically, classic histologic stainings were the only choice, where dyes with different affinities for specific cellular components allowed visualization of the morphogenetic changes. Around the turn of the 19th century, William His Senior pioneered studies on the development of the human heart (34). From that time onward, without almost any exception, the development of the human heart was only studied by the analysis of histologically stained serial sections of staged embryos, along with the preparation of three-dimensional reconstructions. The method of following structures from one section to another and from one developmental stage to the next one is prone to erroneous interpretation, and has resulted in a variety of theories describing the development of virtually every part of the human heart. More recently, labeled antibodies binding to a specific protein or labeled ribonucleic acids hybridizing in situ with specific messenger RNAs, are used for the molecular characterization of the cells making up the developing heart. The staining for protein or mRNA expression by immunohistochemistry or in situ hybridization, respectively, can be done either on serial sections, or on whole embryos. These techniques, together with three-dimensional reconstructions became the most applied tools to visualize spatiotemporal patterns of gene expression in the distinct tissue types of the embryo undergoing rapid morphogenetic changes. Another very important experimental tool for our understanding of cardiac morphogenesis encompasses the irreversible introduction of a traceable molecular marker into the DNA of a mouse in a cell-specific fashion. This makes it possible to follow the fate of the cells originally expressing this marker, and all their derivatives, thus permitting the ability to assess the different cell lineages that contribute to certain structures in the heart
(Fig. 1.4). This technique has been extremely useful to resolve conflicting data regarding the origin of the distinct components of the heart (35).
(Fig. 1.4). This technique has been extremely useful to resolve conflicting data regarding the origin of the distinct components of the heart (35).
TABLE 1.1 Comparison of the Developmental Stages in the Human, Mouse, and Chicken Embryos | ||||||||||||||||||||||||||||||||||||||||
---|---|---|---|---|---|---|---|---|---|---|---|---|---|---|---|---|---|---|---|---|---|---|---|---|---|---|---|---|---|---|---|---|---|---|---|---|---|---|---|---|
|
Formation and Looping of the Heart Tube
Formation of the Embryo
The formation of the primary myocardium and the primitive heart tube is intimately associated with the development of the early embryo itself. After implantation of the blastocyst into the endometrium of the uterus, differentiation rapidly proceeds resulting in a difference between the cells making up the embryo proper (embryoblast) and the surrounding cells making up the future placenta (trophoblast). In the 2nd week of development, the human embryoblast consists of two germ layers, the epiblast (embryonic ectoderm) and the hypoblast (embryonic endoderm). In the 3rd week of development, the proliferation of cells and morphogenetic changes within the epiblast give rise to the entire embryo. Part of the cells making up the epiblast undergoes the process of gastrulation, by which the epiblastic cells lose their epithelial context and migrate through the primitive streak at the embryo’s midline to form consecutively the three embryonic germ layers, endoderm, mesoderm, and ectoderm (Fig. 1.5). At the ectodermal side of the embryonic disc, the amniotic cavity develops, while at the endodermal side the yolk sac forms. This early embryo becomes then in its entirety surrounded by a fluid-filled chorionic cavity, called also extraembryonic coelom, which develops within the loosely arranged extraembryonic mesoderm. The embryonic disc remains linked to the developing placenta through the connecting stalk (Fig. 1.5). Together with the process of gastrulation, the seemingly simple embryonic disc acquires several morphologically and molecularly defined axes of polarity. The dorsoventral axis of the embryo is defined by the ectoderm facing the amnion dorsally versus the hypoblast facing the yolk sac ventrally. The caudocephalic axis is defined by the primitive streak caudally versus the developing neural plate and oropharyngeal membrane cranially. The left–right axis becomes established during gastrulation by leftward movement of the nodal cilia at the most cranial side of the primitive streak. Subsequent to its formation the intraembryonic mesoderm differentiates into the axial notochord, intermediate mesoderm, and lateral plate mesoderm. Concomitantly, the extracellular spaces between the cells of the lateral plate mesoderm unite to form an intraembryonic coelom. The intraembryonic coelom splits the mesoderm into two layers: the splanchnic mesoderm facing the endoderm and the somatic mesoderm leaned against the ectoderm (Fig. 1.6). Cranially and caudally, small round regions of epiblast and hypoblast are not separated from each other and form the oropharyngeal and cloacal membranes, which are the future entrance and exit of the gastrointestinal tract.
Rising the Heart: The Primary Field of Cardiac Progenitors
At the cranial part of the trilaminar embryonic disc a part of the splanchnic mesodermal layer consists of cells destined to differentiate into cardiomyocytes. This part of the lateral plate mesoderm is called the cardiogenic mesoderm, and consists of two populations of cardiac progenitors, the so-called heart fields (36,37,38,39). The epithelial sheath of splanchnic mesodermal cells differentiating first and forming the primitive heart tube makes up the primary heart field. The adjacent pool of cardiogenic cells located central and dorsal to the primary heart field and differentiating at later stages makes up the secondary heart field. Although the primary and secondary heart fields share a common origin (40), they contribute cells to the developing heart in a temporal- and spatial-specific manner (41,42). Studies on single embryonic
stem cell differentiation suggest that the primary cell types of the early heart (myocardial, endocardial, and smooth muscle cells) are derived from a single mesodermal cardiac progenitor (43,44,45). In mouse at approximately embryonic day 7.5 and in human at 2 weeks of development, the primary field of cardiac progenitors becomes arranged at the cranial side of the embryo in a crescent shape. The primary heart field cells are characterized by the expression of several myocardial contractile proteins and “cardiac” transcription factors. Induction of the differentiation of the cardiogenic mesoderm toward the primitive myocardial phenotype occurs by signaling cross talk between multiple tissue types (Fig. 1.7). The transcriptional programs that guide the specification of splanchnic mesoderm into primary myocardium require first the expression of the T-box transcription factor Eomesodermin (46), which at the primitive streak stage directly activates the transcription factor Mesoderm posterior-1 (Mesp1) (47). Mesp1-induced specification of cardiogenic mesoderm is achieved by downregulation of the pluripotency genes and upregulation of “cardiac” transcription factors such as Gata4, Nkx2–5, and enhancer factor Mef2c (48,49). The induced cardiogenic mesodermal cells only acquire a myocardial phenotype when imposed upon by a balance of positive and negative stimuli emanating from the neighboring endodermal, ectodermal, and notochordal cells (Fig. 1.7) (50,51). Signaling from endodermal cells positively regulates cardiac specification through multiple transcription factors and signaling pathways including Sonic hedgehog (Shh), Wnt ligands,
fibroblast growth factors (FGFs), and the members of the transforming growth factor (TGF)-superfamily Nodal and bone morphogenetic proteins (BMPs) (52). Signals from the neural plate, somatic and axial mesoderm, in turn, repress myocardial differentiation (38), mainly through the Wnt signaling pathway. Ligands Wnt11 and Wnt3a in somatic mesoderm as well as BMP inhibitors Noggin and Chordin expressed in the dorsal neural tube and notochord, combinatorially restrict cardiac commitment (53,54).
stem cell differentiation suggest that the primary cell types of the early heart (myocardial, endocardial, and smooth muscle cells) are derived from a single mesodermal cardiac progenitor (43,44,45). In mouse at approximately embryonic day 7.5 and in human at 2 weeks of development, the primary field of cardiac progenitors becomes arranged at the cranial side of the embryo in a crescent shape. The primary heart field cells are characterized by the expression of several myocardial contractile proteins and “cardiac” transcription factors. Induction of the differentiation of the cardiogenic mesoderm toward the primitive myocardial phenotype occurs by signaling cross talk between multiple tissue types (Fig. 1.7). The transcriptional programs that guide the specification of splanchnic mesoderm into primary myocardium require first the expression of the T-box transcription factor Eomesodermin (46), which at the primitive streak stage directly activates the transcription factor Mesoderm posterior-1 (Mesp1) (47). Mesp1-induced specification of cardiogenic mesoderm is achieved by downregulation of the pluripotency genes and upregulation of “cardiac” transcription factors such as Gata4, Nkx2–5, and enhancer factor Mef2c (48,49). The induced cardiogenic mesodermal cells only acquire a myocardial phenotype when imposed upon by a balance of positive and negative stimuli emanating from the neighboring endodermal, ectodermal, and notochordal cells (Fig. 1.7) (50,51). Signaling from endodermal cells positively regulates cardiac specification through multiple transcription factors and signaling pathways including Sonic hedgehog (Shh), Wnt ligands,
fibroblast growth factors (FGFs), and the members of the transforming growth factor (TGF)-superfamily Nodal and bone morphogenetic proteins (BMPs) (52). Signals from the neural plate, somatic and axial mesoderm, in turn, repress myocardial differentiation (38), mainly through the Wnt signaling pathway. Ligands Wnt11 and Wnt3a in somatic mesoderm as well as BMP inhibitors Noggin and Chordin expressed in the dorsal neural tube and notochord, combinatorially restrict cardiac commitment (53,54).
Along with the formation of the trilaminar embryonic disc and the establishment of the primary heart field, the first embryonic vessels also develop. The endothelial cells of the vessels bringing blood to the forming heart tube (future veins), the endocardial cells of the heart tube, and the endothelial cells of the vessels bringing blood away from the heart tube (future aortic arches and dorsal aortas), are initially morphologically identical. The developmental mechanisms underlying their formation and the
expression of molecular markers are, however, different. Thus, in the quail embryo all endothelial cells express the QH1-antigen, whereas only the endocardial cells express two additional extracellular antigens, a fibrillin-like protein and cytotactin (55). In the mouse while the gap junctional protein connexin 37 is expressed in all endothelial cells, connexin 40 only is expressed in the endothelial cells of the arteries, but not in the endocardium or veins (56). The endocardial cells covering the initially bilateral lumens of the forming heart tube are derived from the same cardiogenic mesodermal cells as the primary myocardium (Fig. 1.8) (57,58). In the heart-forming region differentiation of the endothelial cells is induced by local signals from the endoderm and the cardiogenic mesenchyme to form bilateral endothelial tubes that will fuse to form the midline endocardial tube. The initial step of endocardium formation is delamination of the endothelial precursor cells from the precardiac mesoderm. TGF-beta, but not vascular endothelial growth factor (VEGF) appears to mediate this (59). Angiopoietins are known to stimulate endothelial precursor cell migration and proliferation (Fig. 1.8) (60,61). Gata5 is transiently expressed and largely restricted to the endocardial cells in the cardiogenic mesenchyme. Gata5 is induced concomitant with Flt1 and prior to induction of Tie2, ErbB3, and connexin 37 in endothelial differentiation. NFATc is also a transcription factor that is essential for endocardial development, and is not expressed by extracardiac vascular endothelial cells, suggesting that it may cooperate with Gata5 for terminal endocardial cell differentiation (62,63,64). As the endocardial cells become terminally differentiated, several factors are upregulated including epicardin, endothelin-1, tenascin X, and endothelial-specific transcription factor Epas1 (65,66,67).
expression of molecular markers are, however, different. Thus, in the quail embryo all endothelial cells express the QH1-antigen, whereas only the endocardial cells express two additional extracellular antigens, a fibrillin-like protein and cytotactin (55). In the mouse while the gap junctional protein connexin 37 is expressed in all endothelial cells, connexin 40 only is expressed in the endothelial cells of the arteries, but not in the endocardium or veins (56). The endocardial cells covering the initially bilateral lumens of the forming heart tube are derived from the same cardiogenic mesodermal cells as the primary myocardium (Fig. 1.8) (57,58). In the heart-forming region differentiation of the endothelial cells is induced by local signals from the endoderm and the cardiogenic mesenchyme to form bilateral endothelial tubes that will fuse to form the midline endocardial tube. The initial step of endocardium formation is delamination of the endothelial precursor cells from the precardiac mesoderm. TGF-beta, but not vascular endothelial growth factor (VEGF) appears to mediate this (59). Angiopoietins are known to stimulate endothelial precursor cell migration and proliferation (Fig. 1.8) (60,61). Gata5 is transiently expressed and largely restricted to the endocardial cells in the cardiogenic mesenchyme. Gata5 is induced concomitant with Flt1 and prior to induction of Tie2, ErbB3, and connexin 37 in endothelial differentiation. NFATc is also a transcription factor that is essential for endocardial development, and is not expressed by extracardiac vascular endothelial cells, suggesting that it may cooperate with Gata5 for terminal endocardial cell differentiation (62,63,64). As the endocardial cells become terminally differentiated, several factors are upregulated including epicardin, endothelin-1, tenascin X, and endothelial-specific transcription factor Epas1 (65,66,67).
The endothelial cells of the future venous and arterial vessels originate in situ through differentiation of the dorsal paraxial and caudal lateral plate mesodermal cells into clusters of angioblasts, which, with time, increase in size and acquire a lumen filled with the first blood fluids. Such vascular precursors then unite into vessels which become continuous with the vessels of the connecting stalk (68,69). The molecular mechanisms underlying the formation of the first embryonic veins and arteries are not known. It is unclear how the mesodermal cells at exactly determined locations are programmed to differentiate into endothelial cells and to arrange themselves in circular fashion in continuity with one another along the longitudinal axis of the embryo. It is also not known how the lumens within these very first vessels are formed, albeit the morphologic changes are quite well described (70,71).
Formation of the Primitive Heart Tube
Concomitant with the differentiation of the primary heart field the embryo acquires its characteristic curved shape by the process of folding of the initially flat embryonic disc (72). Because the peripheral margins of the embryonic disc hardly grow in contrast to the disc itself, the forming embryo bulges out over the margins, which gives the embryo its shape. By this process also the lateral parts of the embryo are brought together ventrally at the midline. Largely due to the enormous growth of the developing brain and surrounding tissues, the embryo folds in longitudinal direction, by which the forming heart tube and its surrounding coelomic (future pericardial) cavity rotate 180 degrees along the transverse axis and become located ventrally and caudally to the developing brain.
During the lateral folding of the embryo, the myocardial sheets of splanchnic mesoderm steadily bulge into the coelomic cavity. Subsequently they fuse together, forming the bowl-shaped primary cardiac tube being bordered dorsally by the foregut and filled with a gelatinous substance called cardiac jelly (Figs. 1.6 and 1.9) (7,73). In human, it occurs at about 20 days of development. The bowl-shaped myocardial structure continues to bulge into the pericardial cavity until its lateral edges meet dorsally at the midline and fuse to complete the formation of the heart tube. The fusion of the bilateral heart-forming regions and subsequent formation of the embryonic bowl-shaped heart have been demonstrated to depend on the contractile properties of the adjacent endodermal layer (74). The linear heart tube initially remains attached to the dorsal wall of the pericardial cavity by the dorsal mesocardium, which disappears during the process of cardiac looping at later stages, by which the heart tube acquires an S-shape form. The heart tube itself remains attached to the coelomic wall at its arterial and venous poles (Fig. 1.9). Initially, it has been thought that looping of the heart tube simply is the consequence of rapid growth of the tube within a much more slowly expanding pericardial cavity. Experimental studies showed, however, that the heart tube continues to loop even when detached from the dorsal pericardial wall and even loops when the heart no longer beats (75,76). These experiments rule out rapid growth or hemodynamic forces as the driving morphogenetic mechanism. Looping, therefore, seems to be an intrinsic feature of the heart itself, albeit that the exact cause still has to be determined.
Growth of the Primitive Heart Tube and Role of the Secondary Heart Field
Morphologic studies of the early developing heart showed that before initiating the process of looping, the linear heart tube of chicken and human embryos elongates considerably (Fig. 1.10). The speed of morphogenesis in the mouse embryonic heart is so fast that the processes of tube elongation, looping, and even initiation of the chamber formation occur almost simultaneously (77), which is in strong contrast to the human situation. Quantification of cell division activity by measuring bromodeoxyuridine incorporation
into replicating DNA in chicken embryos has demonstrated that the myocardium making up the elongating primitive heart tube hardly proliferates compared to the rapidly dividing cardiac precursors of the secondary heart field and adjacent mesenchyme (78,79,80). In line with these experimental findings, very low levels of the cellular proliferation marker Ki67 were observed in the myocardium of the early human heart tube in contrast to the very high proliferation activity in the coelomic wall. This confirmed that also in human, elongation of the primitive heart tube occurs by addition of cardiomyocytes from outside the heart (Fig. 1.11) (73).
into replicating DNA in chicken embryos has demonstrated that the myocardium making up the elongating primitive heart tube hardly proliferates compared to the rapidly dividing cardiac precursors of the secondary heart field and adjacent mesenchyme (78,79,80). In line with these experimental findings, very low levels of the cellular proliferation marker Ki67 were observed in the myocardium of the early human heart tube in contrast to the very high proliferation activity in the coelomic wall. This confirmed that also in human, elongation of the primitive heart tube occurs by addition of cardiomyocytes from outside the heart (Fig. 1.11) (73).
Although it was already known that the primitive heart tube elongates by addition of cells at its arterial and venous poles while possessing very low proliferative activity in the 1960s and ’70s (78,81,82,83), it took several decades and many experiments to convincingly demonstrate the fact that the atria, RV, and outflow tract are derived from differentiating cells that are added to the heart only after the primitive tube has formed (Fig. 1.12) (84,85,86,87). Recent genetic lineage studies using the Cre-lox technology to mark progenitor cells and their descendants indicate that the primitive heart tube provides only a scaffold that enables a population of cells to be added to the heart during the second phase of differentiation of the cardiac progenitors. While the primitive heart tube is being formed from the myocardializing splanchnic mesoderm, most of the secondary cardiogenic mesoderm is located medially and dorsally (87,88,89,90,91,92,93,94,95,96,97,98,99). These cells remain in a highly proliferating and undifferentiated progenitor state until incorporation into the heart, possibly due to their closer proximity to inhibitory Wnt signals emanating from the ectoderm (Fig. 1.13). Along the course of development, the secondary heart field progenitor cells contiguous with the primitive cardiac tube differentiate into cardiomyocytes, by which the heart tube elongates. Because of this delayed differentiation, these precursors are commonly referred to as the secondary heart field. It should be realized, however, that this terminology only is based on the temporal aspect of two waves of the differentiation of cardiomyocyte progenitors formed from the common mesodermal source (40).
![]() Figure 1.13 Current model of the contribution of the secondary heart field to the definitive heart. A: Depicts schematically the progression from cardiac crescent through the primitive heart tube toward the four-chambered heart. The secondary heart field is located dorsally from the forming heart derived from the primary field. The cells making up the secondary heart field encompass the dorsal pericardial wall, and are added at the venous and arterial poles of the definitive heart. B: Summarizes current knowledge about genetic regulation of proliferation, migration, and differentiation of cardiomyocytes derived from the second heart field. Green arrows indicate positive regulation and red lines suppression. CS, Carnegie stages (of human development); ED, embryonic day (of mouse development), see also Table 1.1. (Modified from Kelly RG. The second heart field. Curr Topics Dev Biol. 2012;100:33–65.) |
Regulation of the differentiation of cardiac progenitors within the secondary heart field, and their survival during elongation of the heart tube involves numerous signaling and transcriptional cascades (Fig. 1.13). These signals both regulate and are regulated by transcription factors in the pharyngeal mesoderm and adjacent cells, including pharyngeal epithelia and neural crest–derived cells, as well as autocrine signals from pharyngeal mesoderm itself (88,89). In the central region of the embryo, the secondary heart field cells are exposed to BMP and noncanonical Wnt signals that drive their differentiation. Pro-proliferation FGF-signaling and prodifferentiation BMP-signaling appear to identify core antagonistic signaling pathways regulating progressive addition of secondary heart field cells to the heart tube (90,91). The balance between these pathways is influenced by neural crest cell invasion of the caudal pharyngeal region that plays a critical role in breaking the proliferative effect of FGF signaling on secondary heart field cells, in addition to a direct later role in septation of the cardiac outflow tract (92,93). Transcription factors such as Islet1 and Tbx1 play central roles in integrating the output of different signaling pathways during secondary heart field development (87,94). Islet1, named after its involvement in pancreatic development, is essential for proliferation and differentiation of secondary heart field cells (ref), but Islet1 expression is extinguished as soon as progenitor cells have differentiated into cardiomyocytes (Fig. 1.11). Lineage studies have shown that the progeny of Islet1-positive cells contribute to most of the heart except the LV (87). A growing number of additional transcription factors from diverse families, including forkhead, homeobox, T-box, GATA, zincfinger, and bHLH factors, have been found to play important roles in secondary heart field development (Fig. 1.13) (89). As such, the pathways regulating the determination and differentiation of secondary heart field cells may provide the foundation for efforts to induce the cardiac lineage from progenitor cells. Furthermore, delineation of the factors that regulate the development of the secondary heart field has revealed differences as compared to the factors governing differentiation of the primary heart field. These
differences, in turn, may be particularly important in deciphering the pathogenesis of congenital defects affecting the arterial pole of the heart.
differences, in turn, may be particularly important in deciphering the pathogenesis of congenital defects affecting the arterial pole of the heart.
Chamber Formation and Ventricular Septation
A fundamental question in cardiac development relates to the establishment of the correct disposition of the chambers and the conduction system (95,96). Whereas the primitive peristaltically contracting heart tube does not need valves, they are essential for the proper functioning of the synchronously contracting four-chambered heart to prevent backflow from a downstream compartment during relaxation and to an upstream compartment during contraction. In the early developing heart, it is not possible to histologically identify the components of the conduction system as one can in the postnatal heart. Despite the absence of a specialized conduction system and valves, the electrical configuration of the embryonic heart consisting of alternating slowly and rapidly contracting segments, as described below, allows the early chamber-forming heart to produce coordinated atrial and ventricular contractions effectively propelling blood forward (97). Because slow conduction is also a prerequisite for nodal function, it may not be coincidental that nodes in these areas have developed, as discussed in Chapter 18.
Development of the Basic Building Plan of the Mammalian Heart
The elongation of the primitive heart tube is followed by the process of looping, during which the ventral part of the tube deviates to the right while bulging more and more ventrally as the ventricular chambers start to develop. At the same time the atrial chambers develop dorsally to the right and left of the forming outflow tract, which leads to the definitive appearance of the human heart at the end of the 8th week of development, when the fetal period begins (Fig. 1.14). Subsequent to looping of the primitive heart tube, it becomes possible to distinguish its outer and inner curvatures (Fig. 1.15). At localized areas of the outer curvature, the primary cardiomyocytes start to proliferate and initiate a genetic program governing their differentiation toward the working myocardium phenotype, which is characterized by the expression of fast-conducting gap-junctional proteins and atrial natriuretic factor
(ANF) (73,98,99). Morphologically this differentiation can be recognized by the rapid expansion of the atrial chambers dorsally and the trabeculated ventricular chambers ventrally, a process that has been called ballooning (98,99). In the human-developing heart, this process was nicely illustrated by Streeter already in the 1940s (100). He demonstrated that the remnants of the smooth-walled primary heart tube persist as a continuous space in between the expanding trabeculated cardiac chambers. This space is bordered by the primary myocardium of the atrioventricular (AV) canal, the inner curvature, and the outflow tract, which flanks the ballooning atrial and the ventricular chambers (Fig. 1.16). This space provides from the very beginning direct communications not only between the developing atria and the respective ventricles, but also between two developing ventricles and the outflow tract (Fig. 1.15C, D). The primary myocardium retains its primitive phenotype characterized by long-lasting contractions and slow conduction, thus fulfilling its temporary valvar and AV delay functions (97,101). After initiation of chamber formation, a new myocardial structure, the systemic venous sinus, is formed at the inflow region of the heart (102,103). Similar to the myocardium of the primary heart tube, the myocardium of the venous sinus initially escapes further differentiation, does not express fast-conducting connexins, and is characterized by high intrinsic automaticity, ensuring dominant pacemaker activity at the inflow of the heart (102,103,104,105,106). At this stage, the basic cardiac building plan, consisting of fast-conducting chambers flanked by slow-conducting myocardium of the venous sinus, AV canal, and outflow tract serves as a blueprint for the formation of the specialized pacemaking and conduction system at appropriate positions, as described in detail in Chapter 18.
(ANF) (73,98,99). Morphologically this differentiation can be recognized by the rapid expansion of the atrial chambers dorsally and the trabeculated ventricular chambers ventrally, a process that has been called ballooning (98,99). In the human-developing heart, this process was nicely illustrated by Streeter already in the 1940s (100). He demonstrated that the remnants of the smooth-walled primary heart tube persist as a continuous space in between the expanding trabeculated cardiac chambers. This space is bordered by the primary myocardium of the atrioventricular (AV) canal, the inner curvature, and the outflow tract, which flanks the ballooning atrial and the ventricular chambers (Fig. 1.16). This space provides from the very beginning direct communications not only between the developing atria and the respective ventricles, but also between two developing ventricles and the outflow tract (Fig. 1.15C, D). The primary myocardium retains its primitive phenotype characterized by long-lasting contractions and slow conduction, thus fulfilling its temporary valvar and AV delay functions (97,101). After initiation of chamber formation, a new myocardial structure, the systemic venous sinus, is formed at the inflow region of the heart (102,103). Similar to the myocardium of the primary heart tube, the myocardium of the venous sinus initially escapes further differentiation, does not express fast-conducting connexins, and is characterized by high intrinsic automaticity, ensuring dominant pacemaker activity at the inflow of the heart (102,103,104,105,106). At this stage, the basic cardiac building plan, consisting of fast-conducting chambers flanked by slow-conducting myocardium of the venous sinus, AV canal, and outflow tract serves as a blueprint for the formation of the specialized pacemaking and conduction system at appropriate positions, as described in detail in Chapter 18.
![]() Figure 1.14 The changing external morphology of the human embryonic heart, as visualized by scanning electron microscopy at consecutive Carnegie stages (CS) of embryonic period of the development, with every stage covering 2 to 3 days of development (Table 1.1). The panels show ventral views of the hearts after removal of the ventral body wall. Note the progressive expansion, or ballooning, of the atrial and ventricular chambers, shortening of the outflow tract and the steady ventral displacement of the RV between stage 11 (∼24 to 25 days) and stage 23 (56 to 58 days). Ao, (ascending) aorta; AVC, atrioventricular canal; LA/RA, left/right atrium; LV/RV, left/right ventricle; OFT, outflow tract; PA, pulmonary artery. (From Oostra RJ, Steding G, Lamers WH, Moorman AFM. Steding’s and Viragh’s Scanning Electron Microscopy Atlas of the Developing Human Heart. New York, NY: Springer; 2007.) |
Despite the great morphologic insights of Streeter, it remained difficult to envision how the developing left and right atria, left and right ventricular chambers, and their respective outlets acquire their correct connections in parallel, as seen in the normal four-chambered heart. This enigma was solved by careful analysis of the expression pattern of a neural tissue antigen Gln2 in the developing human heart. Although the functional significance of the expression of Gln2 in the developing heart is still unknown, this marker was very fruitful for our understanding of the development of the correct AV and ventriculoarterial arrangements (107). Initially, in the early chamber-forming heart, Gln2 expression marks the so-called primary foramen, which is bordered by the right-sided aspect of the AV canal and the crest of the ventricular septum. With ongoing development, the AV canal, marked by the expression of Gln2, expands and shifts rightward, establishing a direct connection between the right atrium (RA) and the RV. A leftward extension of the anterior aspect of the Gln2-positive ring becomes evident at later stages, when the subaortic outflow tract becomes positioned above the LV (Fig. 1.17).
Our understanding of the molecular mechanisms regulating the balance between differentiation into working myocardium, versus inhibition of differentiation and maintenance of the primary myocardial phenotype, has enormously increased over the last decade (Fig. 1.18). One of the earliest events is the activation of the BMP-signaling pathway, with BMP2 as one of the main players (108,109,110). This signaling protein activates the expression of another T-box transcriptional factor, Tbx2, which along with the transcription factors Tbx3 and Msx2 prevents expression of the chamber myocardium-specific gene program, thus protecting the AV canal musculature from further differentiation (111,112,113,114). Another T-box transcription factor, Tbx20, directly interferes with BMP/Smad signaling to suppress Tbx2 expression in the chambers, thereby confining Tbx2 expression to the prospective AV canal region (115). The expression patterns of TBX3 and TBX2 in human embryonic hearts are very similar to those reported in mouse, suggesting conservation of the mechanisms underlying the formation of the chambers and the conduction system (73,116).
Growth and Differentiation of the Left and Right Ventricular Chambers
There are considerable differences in the molecular regulation and morphogenetic processes involved in the development of the RV as opposed to the LV (Figs. 1.19 and 1.20). The cardiomyocytes making up the definitive RV originate from the secondary population of cardiac progenitors, while the LV is a derivative of the primary heart field. Discovery of the secondary heart field led to a reinterpretation of the findings in mice lacking critical regulatory proteins and in transgenic mice harboring enhancers of genes expressed in the heart (117) (Fig. 1.19). The RV was found to be enriched in the transcription factor Hand2, which was shown to be specifically required for expansion of this chamber (118,119,120). This observation further supported the concept that separable regulatory pathways control the development of the ventricles. Right ventricular hypoplasia in mice lacking transcription factor Mef2c, now a known target of Isl1, Gata4, Foxh1, and Tbx20 in the secondary heart field, may, similar to the Hand2 disruption, represent a defect of secondary heart field development (121,122). While Hand2 and the closely related gene Hand1 are both expressed in the progenitor cells of the secondary and primary heart field, Hand1 becomes more enriched in the myocardium of the LV compared to the RV (123). Many of the central transcriptional regulators of cardiac development in the primary heart field, including Nkx2–5 and Gata4, are also found to play an important role in the secondary heart field development (124). The view of an RV being merely derived from the secondary heart field is, however, too simplistic as the zebrafish, while lacking a second (right) ventricle, possesses nonetheless the distinct secondary population of the cardiac progenitors. The secondary field of cardiac progenitors of zebrafish is, similar to mammals, positive for the analogous transcription factors Islet1, Tbx1, and Mef2c and essential for proper development of its two-chambered heart (125,126,127). This reflects the evolutionary changes in the expanded contribution of the secondary mesodermal pool of cardiac progenitors that occurred in higher vertebrates possessing four cardiac chambers. Epigenetic factors, such as the chromatin remodeling, may also contribute to cardiomyocyte differentiation and chamber morphogenesis (128). Protein Smyd1/BOP, disruption of which results in a small right ventricular segment and poor development of the left ventricular myocardium, is a direct target of Mef2c and contains a MYND domain that recruits histone deacetylase (128,129). This suggests that a transcriptional cascade involving Isl1, Mef2c, Smyd1/BOP, and Hand proteins regulates the development of ventricular cardiomyocytes. Furthermore, a direct role for several histone deacetylases in cardiac development was also demonstrated by a failure of ventricular growth in compound mutant mice lacking the isoforms 5 and 9 of
this protein (130). These and other pathways in the heart appear to be regulated by a member of SWI/SNF chromatin remodeling complex Baf60c, a transcriptional cofactor enriched in fast-twitch muscle (131). This suggests further that transcriptional activity of cardiac DNA–binding proteins is highly regulated through epigenetic events.
this protein (130). These and other pathways in the heart appear to be regulated by a member of SWI/SNF chromatin remodeling complex Baf60c, a transcriptional cofactor enriched in fast-twitch muscle (131). This suggests further that transcriptional activity of cardiac DNA–binding proteins is highly regulated through epigenetic events.
Experimental studies in chicken and mouse embryos revealed that along with the proliferation of the cardiomyocytes making up the free walls of ventricular chambers, the contributions by differentiating cells from outflow tract and AV canal myocardium also play an important role in the expansion process (Fig. 1.20). Labeling experiments have shown that while cells from the secondary heart field form the distal outflow tract, the cardiomyocytes of its proximal part differentiate into the working phenotype, forming the free wall of the RV (82,132). In the mouse, genetic lineage studies of Tbx2-positive AV canal myocardium demonstrated that the primary cardiomyocytes at the transition zone between the AV canal and left ventricular wall undergo a comparable process of differentiation and displacement to form the larger part of the free wall of the LV (133). The cells added to the forming free walls of the ventricles obey the ventricular program of gene expression and have much higher proliferation rates in comparison with the flanking regions of AV canal and outflow tract (Fig. 1.20). Expression of TBX2 in the AV canal myocardium of the early human chamber-forming heart decreased into ventricular direction (73), which resembled the expression pattern in the mouse, where the Tbx2-lineage has been shown to contribute to the left ventricular free wall (133). It is tempting to speculate, therefore, that impairment of this contribution to the ventricle may result in left ventricular hypoplasia.
Soon after starting of ballooning, the developing ventricular chambers acquire initially tiny, but rapidly elongating trabeculations at their inner surface. As development proceeds, a compact
layer of myocardium at the outer free wall is formed (9,10). The trabeculations within both ventricles express the rapid conduction connexins 40 and 43 and provide the precursor cells for the Purkinje network of the ventricular conduction system (56,73,111), as described in Chapter 18. During normal heart development, proliferation ceases in the trabeculations soon after their formation, while the outer ventricular wall becomes highly proliferative to form the compact myocardial layer (73,77,134), thus meeting the increasing demand to produce more powerful contractions. Unlike the trabecular myocardium, the newly formed compact layer of the ventricular wall does not express connexin 40. The extent of the trabeculations within the ventricular chambers does not change significantly during development compared to the enormous growth of the compact layer (Fig. 1.21). This suggests that failure of proper formation of the compact ventricular wall and abnormal continuing growth of the trabecular layer are responsible for the so-called noncompaction cardiomyopathy, where the extensive trabecular network coexists with a compact layer of decreased thickness (135).
layer of myocardium at the outer free wall is formed (9,10). The trabeculations within both ventricles express the rapid conduction connexins 40 and 43 and provide the precursor cells for the Purkinje network of the ventricular conduction system (56,73,111), as described in Chapter 18. During normal heart development, proliferation ceases in the trabeculations soon after their formation, while the outer ventricular wall becomes highly proliferative to form the compact myocardial layer (73,77,134), thus meeting the increasing demand to produce more powerful contractions. Unlike the trabecular myocardium, the newly formed compact layer of the ventricular wall does not express connexin 40. The extent of the trabeculations within the ventricular chambers does not change significantly during development compared to the enormous growth of the compact layer (Fig. 1.21). This suggests that failure of proper formation of the compact ventricular wall and abnormal continuing growth of the trabecular layer are responsible for the so-called noncompaction cardiomyopathy, where the extensive trabecular network coexists with a compact layer of decreased thickness (135).
Ventricular Septation
The interventricular septum in the formed heart is composed of a small membranous part and a much larger muscular part, which largely contributes to the formation of the ejection force of the LV during systolic contraction of the definitive heart. Little is known about the morphogenetic events that lead to the formation of the muscular component of the ventricular septum. General belief is, however, that when two ventricles are formed by ballooning from the primitive heart tube, the small part between them does not follow the chamber myocardial gene program, and do not balloon. Interestingly, during the very first stages of chamber formation in the human embryonic heart, a considerable part of the primitive heart tube persists between the ballooning RV and LV, thus forming a canal-like communication rather than a real septum (Fig. 1.22) (11). This situation is markedly different from that seen in the embryonic mouse heart, where from the onset of chamber formation a tiny ventricular septum is evident between the developing ventricular chambers (136). From the very beginning, the canal-like communication between the ventricles in the human embryonic heart is dorsally bordered by the primary myocardium of the inner curvature. Therefore, although often misleadingly and inappropriately named as interventricular, this communication is in fact just a part of the original lumen of the primary heart tube. In chamber-forming heart, this original lumen becomes a primary foramen, a centrally located free space. As already emphasized above, the primary foramen from the very beginning secures direct communications between the developing atria and the respective ventricles, and between the developing ventricles and the developing outflow tract. While the expansion of the ventricular chambers in the human embryo proceeds, the wall bordering the initial canal-like communication in between becomes the crest of the ventricular septum. There is a discrepancy in the rate of cell proliferation within the rapidly growing ventricular free walls and that in the slowly growing ventricular septum (Fig. 1.23) (137). Already in 1906, Sir Arthur Keith remarked that the lagging behind of the part of the primary heart tube between the two ballooning ventricles is the mechanism by which the ventricular septum, carrying the bundle of His, is formed (138). Experiments with transgenic mice lacking the transcription factors Hand1 or Hand2, which are essential for the proper formation of either the left or right ventricular chambers, showed that in the case of failed expansion of one of the ventricles the septum in between develops insufficiently (119,123).
The contribution of the molecularly left versus right ventricular myocardium to the definitive muscular ventricular septum was
analyzed using two transgenic mouse lines having complementary expression patterns of the lacZ reporter gene driven by the LV-specific MLC3f and RV-specific MLC1v genes (Fig. 1.23) (139). Between embryonic days 9.5 and 11.5, the developing muscular ventricular septum shows symmetric left and right ventricular identity. From embryonic day 12.5 onward, MLC1v-lacZ negative myocytes with a left ventricular identity dominate the ventricular septum, supporting the notion of its particular role of supporting the pump function of the LV. Furthermore, transgenic tracing experiments of Tbx2-positive primary cardiomyocytes suggest that the precursors for the ventricular septum originate from the bowl-shaped primitive heart tube, whereas the free left ventricular wall is formed from the differentiating cells of the adjacent AV canal as described above (133). The transcription factor Tbx18 is expressed in the myocardium of the left ventricular free wall and left side of the developing ventricular septum, providing additional support for the presence of left and right ventricular identities within the septum (139,140).
analyzed using two transgenic mouse lines having complementary expression patterns of the lacZ reporter gene driven by the LV-specific MLC3f and RV-specific MLC1v genes (Fig. 1.23) (139). Between embryonic days 9.5 and 11.5, the developing muscular ventricular septum shows symmetric left and right ventricular identity. From embryonic day 12.5 onward, MLC1v-lacZ negative myocytes with a left ventricular identity dominate the ventricular septum, supporting the notion of its particular role of supporting the pump function of the LV. Furthermore, transgenic tracing experiments of Tbx2-positive primary cardiomyocytes suggest that the precursors for the ventricular septum originate from the bowl-shaped primitive heart tube, whereas the free left ventricular wall is formed from the differentiating cells of the adjacent AV canal as described above (133). The transcription factor Tbx18 is expressed in the myocardium of the left ventricular free wall and left side of the developing ventricular septum, providing additional support for the presence of left and right ventricular identities within the septum (139,140).
Complete closure of the primary foramen on top of the interventricular septum would result in a blockade of the left ventricular outlet to the aorta, which would be incompatible even with intrauterine life. The primary foramen above the broad muscular septum is instead remolded into the subaortic outlet of the LV and the inlet of the RV (8,9). Subsequent to the rightward movement of the entire AV canal, the right-sided margins of the central AV cushions become positioned above the cavity of the RV. By the subsequent fusion of the AV cushions, this mesenchymal mass forms the dorsal wall of the primary foramen. The communication between the left ventricular outflow tract and the right ventricular cavity is closed then by the fusion of the right-sided margins of the AV cushions with the ridges within the proximal outflow tract (Fig. 1.24) (8,141,142). In the human embryonic heart, this occurs at the end of 7th week of the development (143,144). During the early fetal period, the remodeling of these fused mesenchymal tissues together with the delamination of the septal leaflet of the tricuspid valve will produce the membranous part of the ventricular septum, separating thus the left ventricular outlet from the right ventricular inlet. In normal human hearts, the membranous septum is the only structure present between the left ventricular outflow tract and the cavity of the RV with no real outlet ventricular septum existing. In many hearts with outlet-type ventricular septal defects, outflow tracts themselves are often malformed, and there is a clearly discernable and malaligned muscular septal structure located between the outlets to the arterial valves (145). As described below, the cardiac neural crest–derived cells are crucial for both the normal formation of the cardiac outflow tract and its septation; hearts with so-called conotruncal malformations typically have outlet-type ventricular septal defects with a malaligned muscular outlet ventricular septum. This occurs probably due to defective fusion of the mesenchymal tissues of the outflow tract with those of the AV canal.
Development of the Venous Pole of the Heart
The venous pole of the human heart has a complex morphology reflecting its long evolutionary history. It comprises the union of the caval veins with the systemic venous sinus part of the RA, and the connection of the pulmonary veins to the left atrium (LA) with the atrial septum in between (146,147). In the postnatal heart, the RA consists of a smooth-walled component, called the sinus venarum receiving the caval veins, and a large trabeculated appendage. Both components of the RA are derived from distinct progenitor populations and undergo distinct pathways of differentiation. The LA is composed of a large smooth-walled component receiving the pulmonary veins and a small finger-like trabeculated appendage. Albeit both components of the LA develop from different embryonic structures, they seem to undergo a comparable process of myocardial differentiation. Descriptions of the development of the venous pole for a long time remained controversial not only because of difficulties in describing the rapidly occurring changes in morphology, but also because of the inconsistent use of terminology, with the same structures described in different words by different investigators. Lineage studies in experimental animals and gene expression analyses in human embryos allowed genetic distinction of the different component parts of the atrial chambers, and permitted accurate descriptions of the boundaries between the bodies of the atrial chambers, their appendages and vestibules, and the systemic and pulmonary venous tributaries (102,103).
Morphogenesis and Differentiation of the Atria
At the early looping phase of the primitive heart tube, the venous pole of the heart is nearly symmetric. Neither morphologically nor molecularly is it possible to identify the atria or the venous sinus. At about 24 days of development in human, when the looping process is almost complete and chamber formation is initiated, the small bulging structure at the dorsal side of the caudal part of heart tube is identifiable as the future common atrium, but still without a discernable venous sinus (Fig. 1.9). The two pouches of the working myocardium balloon out in parallel from the newly formed atrial part of the primary tube to form the atrial appendages, one to the right and the other to the left of the developing arterial pole (Fig. 1.14). A large part of the atrial myocardial walls is formed by the cardiomyocytes derived from a caudal population of secondary heart field cardiac progenitors (87,148,149,150,151). Distinct aspects of atrial as compared to ventricular gene expression appear to be in part regulated by Irx4, a member of the Iroquois family of transcription factors (152). Furthermore, Gata6, a canonical Wnt signaling targeting transcription factor, is required in atrial progenitor cells. The proliferation of caudal secondary heart field progenitor cells forming the atrial chambers was shown to be reduced in transgenic mice lacking Wnt2, which results in failure of atrial and AV septation (153). As at the arterial pole, distinct subpopulations of secondary heart field progenitor cells have been identified, such as those giving rise to sinus venosus, pulmonary vein myocardium, and septal or nonseptal atrial myocardium (151,154,155). The identity of the myocardium of the morphologically RA as opposed to the morphologically LA is established by the expression of genes responsible for left–right asymmetry (156,157). In the absence of Pitx2c, one of the best known left–right asymmetry regulators, in the transgenic mouse, the heart develops two morphologically right appendages, along with bilateral sinus nodes (158). Interestingly, the cardiac phenotype of human PITX2 gene mutations, described as Rieger syndrome, is much milder and does not involve disorders in left–right lateralization at all (159).
In molecular terms, the primary myocardium can be distinguished from the myocardium that forms the walls of the atrial pouches by the expression of connexin 40 and ANF, which are never expressed in the primary myocardium (Fig. 1.25) (56,98,103,104). Interestingly, in the mammalian developing heart the myocardial dorsal wall of the common atrium surrounding the dorsal
mesocardium which contains the orifice of the common pulmonary vein is positive for connexin 40, but negative for ANF (104) (Fig. 1.26). This part of the atrial wall, dubbed mediastinal myocardium is the result of continuous differentiation of the caudal secondary heart field being added to the heart through the dorsal mesocardium, and is most probably related to the development of the pulmonary circulation. At later stages, the myocardium of the developing primary atrial septum is also connexin 40 positive but ANF-negative, reflecting its origin from the mediastinal myocardium.
mesocardium which contains the orifice of the common pulmonary vein is positive for connexin 40, but negative for ANF (104) (Fig. 1.26). This part of the atrial wall, dubbed mediastinal myocardium is the result of continuous differentiation of the caudal secondary heart field being added to the heart through the dorsal mesocardium, and is most probably related to the development of the pulmonary circulation. At later stages, the myocardium of the developing primary atrial septum is also connexin 40 positive but ANF-negative, reflecting its origin from the mediastinal myocardium.
At early stages of chamber formation, the common atrium is almost exclusively connected to the LV through a discrete AV canal, which is positioned to the left of the midline. Only after initiation of the ballooning of the atrial chambers, another new structure is added to the caudal pole of the developing heart, the venous sinus
(102,103,104). In the human embryonic heart, the systemic venous sinus is first discernible at the end of 4th week of development as a small hollow structure caudally to the common atrial chamber (Fig. 1.24). Initially, the venous sinus has nonmyocardial walls expressing Tbx18 and HCN4, and is no more than the confluence of the systemic venous channels entering the heart in relatively symmetrical fashion (103,104). Only after formation of the atria, the mesenchymal walls of the venous sinus become myocardial. The myocardium of the venous sinus is derived from Nkx2-5-negative and Tbx18-positive progenitor cells, proliferation of which also is dependent on canonical Wnt-signaling (102,160). It has from the onset a genetic program that is distinct from that of the atrial working myocardium, and initially retains the characteristics of high automaticity and slow conduction. Concomitant with the myocardialization of the venous sinus walls, the connection of the venous sinus becomes confined to the right side of the common atrium. In human, it occurs during the 5th week of development (103,161,162). At the boundary between venous sinus and the RA myocardial folds, the so-called venous valves develop. The left venous valve is regressed completely at birth, while the right one remains as the Eustachian valve guarding the orifice of the inferior caval vein and directs, during fetal life, oxygenated blood coming from the venous duct toward the oval interatrial foramen (163). The lateral wall of the venous sinus becomes thickened and remains strongly positive for HCN4, being the primordium of the sinus node (Fig. 1.26), as described in detail in Chapter 18.
(102,103,104). In the human embryonic heart, the systemic venous sinus is first discernible at the end of 4th week of development as a small hollow structure caudally to the common atrial chamber (Fig. 1.24). Initially, the venous sinus has nonmyocardial walls expressing Tbx18 and HCN4, and is no more than the confluence of the systemic venous channels entering the heart in relatively symmetrical fashion (103,104). Only after formation of the atria, the mesenchymal walls of the venous sinus become myocardial. The myocardium of the venous sinus is derived from Nkx2-5-negative and Tbx18-positive progenitor cells, proliferation of which also is dependent on canonical Wnt-signaling (102,160). It has from the onset a genetic program that is distinct from that of the atrial working myocardium, and initially retains the characteristics of high automaticity and slow conduction. Concomitant with the myocardialization of the venous sinus walls, the connection of the venous sinus becomes confined to the right side of the common atrium. In human, it occurs during the 5th week of development (103,161,162). At the boundary between venous sinus and the RA myocardial folds, the so-called venous valves develop. The left venous valve is regressed completely at birth, while the right one remains as the Eustachian valve guarding the orifice of the inferior caval vein and directs, during fetal life, oxygenated blood coming from the venous duct toward the oval interatrial foramen (163). The lateral wall of the venous sinus becomes thickened and remains strongly positive for HCN4, being the primordium of the sinus node (Fig. 1.26), as described in detail in Chapter 18.
Atrial Septation
In the human-developing heart during the 5th week of development, the common atrium starts to divide into two distinct chambers by formation of the primary atrial septum (Figs. 1.25 and 1.27). Before the primary atrial septum starts to form, a groove is visible on the outer surface of the common atrium dividing it into left and right halves. On the inner surface, this groove remains covered by the remnants of the cardiac jelly, an acellular substance produced by the primary myocardium of the primitive heart tube. Subsequently, this jelly becomes populated by mesenchymal cells derived from the covering endocardial cells by a process called endothelial-to-mesenchymal transformation (EMT). The newly formed mesenchyme on the wall of the dividing common atrium has been suggested to interact with the adjacent cardiomyocytes,
resulting in local activation of cellular proliferation leading to the formation of the thin falciform myocardial sheet, the primary atrial septum (164,165). The left half of the common atrium expresses gene Pitx2c, which in the mouse is essential for the proper development of the morphologically left cardiac structures (149,166). Interestingly, even the dorsal wall of the morphologically RA, being formed by the mediastinal myocardium, and the myocardium of the primary atrial septum are also Pitx2c-positive. As the atrial septum is not formed in Pitx2c knockout mice, this suggests that the development of the atrial septum is closely related to the development of the LA.
resulting in local activation of cellular proliferation leading to the formation of the thin falciform myocardial sheet, the primary atrial septum (164,165). The left half of the common atrium expresses gene Pitx2c, which in the mouse is essential for the proper development of the morphologically left cardiac structures (149,166). Interestingly, even the dorsal wall of the morphologically RA, being formed by the mediastinal myocardium, and the myocardium of the primary atrial septum are also Pitx2c-positive. As the atrial septum is not formed in Pitx2c knockout mice, this suggests that the development of the atrial septum is closely related to the development of the LA.
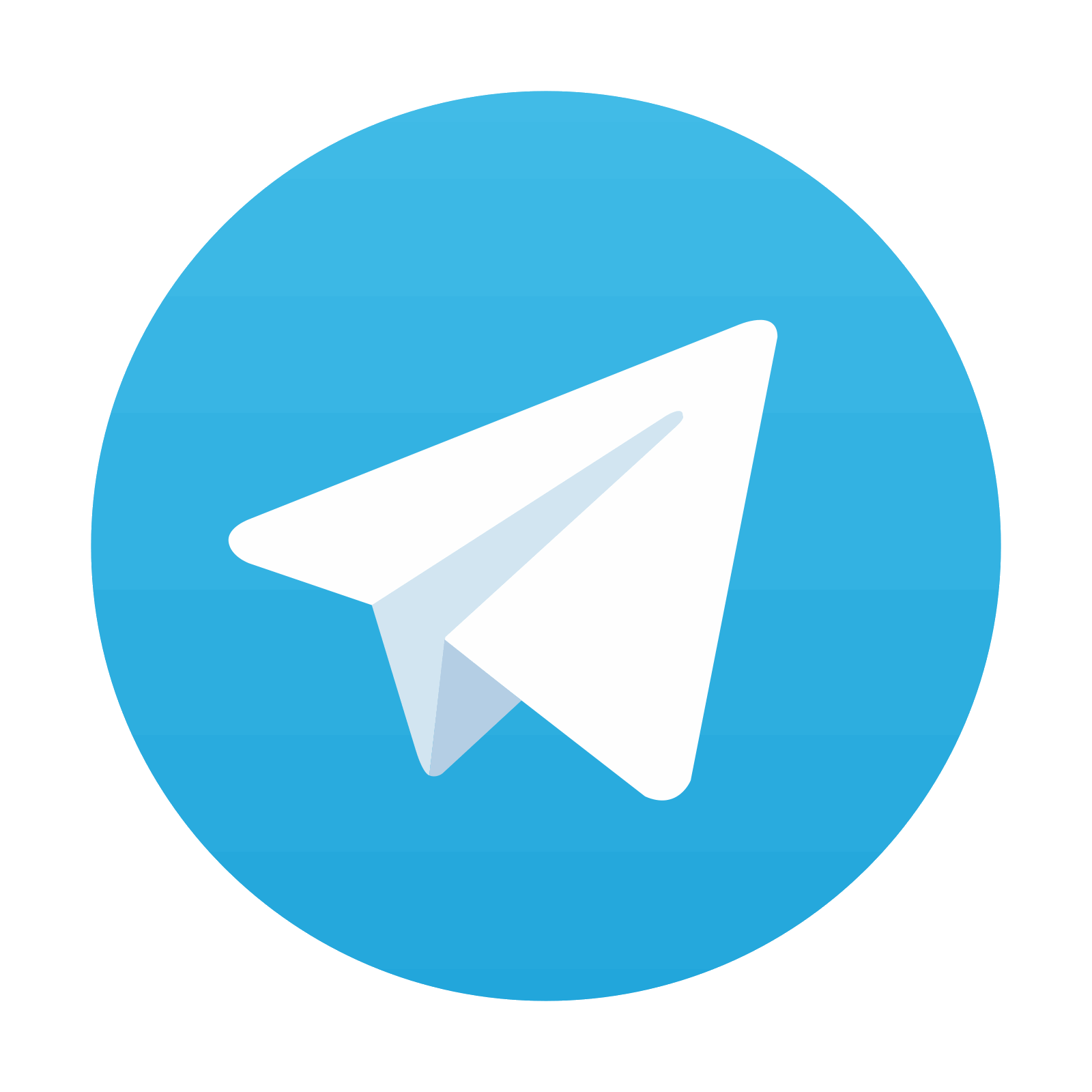
Stay updated, free articles. Join our Telegram channel
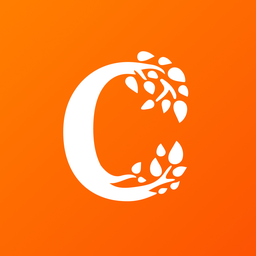
Full access? Get Clinical Tree
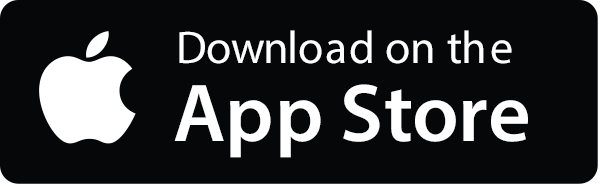
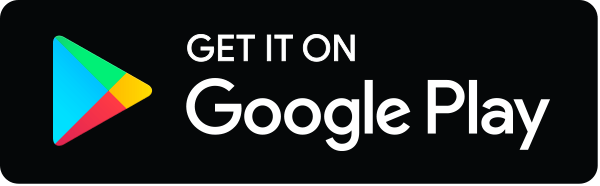