Ram Kumar Subramanyan1, Robert H. Anderson2, and Peter J. Gruber3 1Children’s Hospital of Los Angeles, Los Angeles, CA, USA 2Institute of Genetic Medicine, Newcastle upon Tyne, UK 3Yale New Haven Children’s Hospital, New Haven, CT, USA Major advances in molecular genetics, establishment of appropriate animal models, and improvements in analytical techniques have contributed to a greater understanding of cardiac development. Modern cardiac embryology now combines molecular and cellular biologic techniques with traditional embryologic morphologic approaches across multiple model systems. A significant proportion of our understanding continues to be derived from nonhuman experimental models, supplemented by observations imputed from the congenitally malformed human heart [1]. In early studies, avian embryos were the favored experimental model because of the ease with which they could be observed and manipulated. Due to the strength of genetic and molecular investigative tools, the mouse has now become the preferred model for studying cardiac development. Table 1.1 provides a simplified comparison of developmental staging in human, mouse, and chicken embryos [2–8]. Understanding cardiac development not only has implications for classifying and managing congenital heart disease, but also provides a platform for the development of novel management approaches, in both children and adults. With a goal of simplifying the description of complex developmental structures, in this chapter we have made efforts to harmonize nomenclature using descriptive terms that relate as much as possible to human development. Thus, “anterior‐posterior axis” is replaced by “dorsal–ventral axis” or “cranial–caudal.” “Anterior” is often replaced by “ventral” or “cranial.” “Posterior” is frequently replaced by “dorsal” or “caudal.” “Conus” is replaced by “proximal outflow tract,” and “truncus” is replaced by distal outflow tract. The “dorsal mesocardial protrusion” is referred to as the “vestibular spine.” All cells destined to become part of the heart derive from populations of undifferentiated precursors. These precursor cells are influenced by external signals and guided to their final developmental state. In humans, during the second week following fertilization, the blastocyst has partially embedded into the uterine endometrium. At this stage, the inner cell mass, or embryoblast, differentiates into two distinct layers of cells: a larger columnar epiblast layer and the smaller cuboidal hypoblast layer. The third week of development is characterized by the next critical embryonic process, termed gastrulation. A primitive streak is formed in the epiblast layer, following which some epiblast cells invaginate under and displace the hypoblast. Subsequent widespread cell migration into, and reorganization within, the blastocele cavity results in the formation of three germ layers: the ectoderm, mesoderm, and endoderm. This sets the stage for the determination of the future body plan of the embryo (Figure 1.1) [3, 9]. Migrating epiblast cells, which have now formed the mesoderm of the embryo, gradually travel cephalad. During this migration, they join the lateral plate mesoderm at the level of the primitive node. The lateral plate mesoderm then divides into two layers: a splanchnic layer directly above the endoderm, and a somatic layer directly below the ectoderm. The anterior endoderm provides signals to splanchnic mesodermal cells to enter the precardiac lineage. Fibroblast growth factors (FGFs)‐1, ‐2, and ‐4, and bone morphogenetic protein 2 (BMP‐2), are proteins that appear to be critical to this process [10]. To date, however, no single gene has been identified whose ablation leads to a specific failure of all myocardial differentiation from precardiac mesoderm. It is reasonable to speculate that such lack of reliance on one gene was likely gained as an evolutionary advantage. This could be the result of either (i) considerable genetic redundancy in precardiac myocyte differentiation, or (ii) an unsuspected diversity of precardiac myocyte lineages following independent genetic pathways. By this time in development, precardiac cells begin to express a variety of specific molecular markers, such as the transcription factors NKX2‐5, MEF2, HAND1, HAND2, GATA4, TBX5, and ISL1 [11–17]. The region of splanchnic mesoderm expressing precardiac markers is also known as the “heart‐forming field.” It is larger than the region that will eventually contribute cells to the heart tube [18]. In rodent embryos, precardiac mesodermal cells exhibit spontaneous contractile activity, indicating a relatively advanced state of differentiation toward the cardiac myocyte lineage [19, 20]. Table 1.1 Simplified comparison of developmental stages between human, mouse, and chicken embryos. AV, atrioventricular; OFT, outflow tract. The precardiac mesodermal cell mass continues to migrate cephalad as a coalesced single unit, rather than as a collection of independent cells. Ultimately, they pass on either side of the prochordal plate to finally converge in the midline cranial to the intestinal portal. Following their convergence, the total premyocardial cell population forms a horseshoe‐shaped crescent called the first, or primary, heart field. The cues that enable and promote movement of these cells are provided by a noncardiac tissue, the endoderm, as demonstrated by experimental removal of the endoderm and/or ectoderm [10]. The extracellular matrix molecule fibronectin may be one of the important components of the endodermal surface to which the precardiac cells are responding [21]. Precursors of the endocardium follow similar migratory pathways as the precardiac cells, but with important differences. Pre‐endocardial cells and pre‐endothelial cells are known as angioblasts. The endocardial angioblasts are first detectable in the splanchnic mesoderm. Mesodermal cells are induced to enter the angioblast lineage by signals such as transforming growth factor beta (TGFβ) 2–4 and vascular endothelial growth factor (VEGF) signaling from the endoderm [10]. Endocardial angioblasts migrate anteriorly and to the midline with the premyocardial cell mass, but they do so as individual cells. At this point in cardiac organogenesis, the developing heart has not yet obtained its full complement of cell populations necessary for development. Based on early fate‐mapping studies [22–24], the primary bilateral fields of precardiac mesoderm that form the early heart structure are collectively called the first heart field. This precardiac mesoderm was long considered the precursor tissue of the heart. Further studies then showed that growth of the heart tube, specifically at the arterial pole, depended on the addition of cardiac tissue from a secondary pool of progenitor cells [25]. It was not until the early twenty‐first century that the nature of this additional cell population, called the second heart field, was finally elucidated. The combined studies of various laboratories [26–30] have provided significant new insights into the importance of this additional population of cells regarding the elongation and growth of the heart tube, the outflow tract development, and the formation of a mature four‐chambered heart (Figures 1.2 and 1.3) [3]. This second cardiogenic field of cells is located dorsal and caudal to the wall of the developing pericardial cavity, and lies contiguous with and medial to the primary cardiac crescent. Immunohistochemical, in situ hybridization, and cell fate‐tracing techniques have demonstrated that the outflow tract primordia, the right ventricle, much of the muscular ventricular septum, and parts of the cardiac venous pole develop from the second heart field [29, 31]. Figure 1.1 Simplified schema of gastrulation, precardiac cell migration, and formation of the heart‐forming fields. (A) Cells destined to become cardiac cells migrate from the epiblast into the primitive streak through a broad region caudal to the most anterior portion of the primitive streak. The direction of migration of the gastrulated cells, as indicated by the arrows, is away from the midline and anteriorly on each side. (B) The embryo in cross‐section at the level indicated by the dotted line in (A). The precardiac mesoderm forms an epithelial sheet closely associated with the endoderm. The pre‐endocardial cells are scattered throughout the same region and can be distinguished immunohistochemically from the general precardiac mesoderm. (C, D) Two lateral precardiac mesoderm populations (also known as heart‐forming fields) migrate cranially before turning toward the midline (C). They meet in the midline, as shown in (D), at a location immediately cranial to the anterior intestinal portal. Source: McQuinn T, Wessels A 2003 / With permission of Elsevier. Figure 1.2 Formation of the heart tube is initiated by fusion of the bilateral precardiac mesoderm populations in the midline, resulting in formation of a myocardial tube surrounding an endothelial (endocardial) channel. The myocardial population of the cardiac tube at this stage consists of only the precursors of the future trabeculated portions of the left ventricle. Additional segments are added by ongoing migration of precardiac mesoderm into the heart tube. Source: Reproduced by permission from McQuinn T, Wessels A, in Pediatric Cardiac Surgery, 3rd ed. Philadelphia, PA: Mosby; 2003, pp. 1–24. A developmentally distinct, third set of cells, the neural crest, contributes to the ultimate structure of the heart. These arise from the ectodermal neural tube. In vertebrate development, the neural crest is a transient structure originating in the most dorsal region of the neural tube [32, 33]. The cells of the neural crest reside on the lateral margins of the neural plate. These right‐ and left‐sided populations are brought into apposition by the folding of the neural plate into the neural tube. The cells then disperse and migrate to multiple destinations. As with closure of the neural tube, this process is initiated cranially and extends caudally [9]. Specific regions of the neural crest seed cells to specific structures [34]. The region of the neural crest contributing to cardiac and fourth aortic arch morphogenesis is often referred to as the “cardiac” neural crest [9, 32]. Cells from the cardiac neural crest migrate along the third, fourth, and sixth aortic arch vessels to reach the developing heart. These cells are necessary for aortopulmonary septation, outflow tract septation, and formation of the tunica media of the third, fourth, and sixth aortic arch vessels [32, 35]. An additional population of neural crest cells differentiates into the entirety of the autonomic nervous system of the heart [36]. Other neural crest–derived structures in proximity to the developing heart and great vessels include the thymus, thyroid, and parathyroid glands [37, 38]. Figure 1.3 Normal heart development requires integration of cell populations from multiple sources. (A) Precardiac mesoderm gives rise to the endocardium and most cardiac cushion cells. Premyocardial cells give rise to the entire spectrum of cardiac myocyte phenotypes. The primary heart‐forming field will give rise to most of the myocardium of the atria and left ventricle. The anterior heart‐forming field will give rise to the myocardium of the outflow tract, right ventricle, and interventricular septum. (B) Multiple extracardiac embryonic tissues provide critical cell populations to normal cardiac development. These cell populations include cardiac neural crest cells as well as cells from the proepicardium and the dorsal mesocardium. Source: McQuinn T, Wessels A 2003 / With permission of Elsevier. Last, an epicardial layer of the heart develops from a histologically and functionally complex precursor tissue called the proepicardium, or proepicardial organ [39]. This is a transient cluster of cells that arise as a mesothelial outgrowth ventral and caudal to the developing heart. Soon after formation, the proepicardium migrates away from the body wall, with its cells extending over the surface of the heart to give rise to the epicardium. These cells eventually flatten over the surface of the myocardium, and develop morphologic characteristics compatible with primitive epithelial cells. The flattening process also causes cells to occupy a greater surface area, bringing adjacent clusters of cells into contact with each other until a continuous sheet results. A subset of the proepicardial cells invades the underlying heart tube and contributes to nonmyocardial mesenchymal cells such as fibroblasts and smooth muscle cells of the coronary arteries. In the mouse, where the process is well studied, these events occur in mid‐gestation, although in the human they likely occur earlier [39, 40]. Building on prior work, more recent studies suggest an important role for the epicardium in the diversification of cardiac lineages and potentially as a source of progenitor cell populations [41, 42]. The initial cardiac crescent is located cranial to the prochordal plate. With closure of the neural tube, there is rapid cephalad growth of the central nervous system such that it extends over and around the developing heart. This leads to a cephalocaudal flexion of the embryo, bringing the cardiogenic plate ventral and caudal to the prochordal plate. At approximately the same time, the embryo folds in a transverse plane, bringing onto close apposition the lateral tubes of endothelial cells that were formed from early angioblasts. These tubes then fuse in a cephalocaudal direction to form the primitive single endocardial heart tube. Soon after the formation of the cardiac tube, the heartbeat is initiated and blood circulation can be observed. These events take place at embryonic day 8.5 in the mouse, and day 20 in the human. With the initiation of circulation, the heart becomes the first organ to adopt its essential mature function in the embryo. The heart tube at the time of its formation is connected to the foregut along its dorsal surface throughout its length by a structure called the dorsal mesocardium [43]. As cardiac looping proceeds, the dorsal mesocardium degenerates until it remains connected only at the atrial and arterial poles of the heart. The disintegration of the central portion of the dorsal mesocardium is a key event for looping to proceed normally, while the arterial and venous attachments provide “anchors” for the looping heart tube. A dorsal mesenchymal protrusion, described centuries earlier as the “spina vestibule” or vestibular spine [44], extends into the atrial walls through the rightward margin of the persisting dorsal mesocardium, and is likely a derivative of the second heart field [28, 45]. An important pathway for cellular migration, it contributes to atrioventricular (AV) septation, and serves as a scaffold for entry to the atria of the developing pulmonary veins [46, 47]. At the time the heartbeat is initiated, the heart tube primarily consists of cells from the first heart field that form the future left ventricular tissues [48, 49]. Cells that form the outflow tract, the right ventricle [25], the AV junction [48], the atria, and the systemic venous sinus, also known as the sinus venosus, are added to the heart as looping proceeds [50]. In prelooping and early looping stages the primitive heart tube consists of circumferential sheets of myocardial cells 2–3 layers thick surrounding the inner endothelial tube. These layers are separated by an acellular matrix–rich space known as the cardiac jelly. As looping continues, the future segments can be distinguished morphologically by their position in the heart tube, and by structural features, such as the concentration of cardiac jelly in the AV canal and outflow segments. Segments can be distinguished physiologically by measurement of the differences in their velocity of muscular contraction and relaxation, their rates of spontaneous pacemaker activity, and the speed of electrical impulse conduction [51, 52]. Segmental differentiation creates the physiologic competence of the embryonic heart [53]. Unidirectional antegrade blood flow is maintained by organization of the heart tube into alternating regions of rapid and slow contractile properties [54]. The atrium has the fastest rate of spontaneous contractility, and is the site of pacemaker activity. A wave of depolarization spreads from myocyte to myocyte from the atrium to the outflow tract, but the velocity of conduction is not uniform throughout the length of the heart tube. Atrial conduction is rapid, AV conduction is slow, ventricular conduction is rapid, and outlet conduction is slow. The zones of rapid conduction show rapid contraction–relaxation mechanical properties, while the slow zones of conduction demonstrate slow, sustained contractions. The result is a forceful contraction of the atria, followed by a sphincter‐like contraction of the AV junction. Prior to maturation of AV valves, these contractions prevent the retrograde flow of blood during the forceful ventricular ejection phase. The cardiac cycle of the heart tube is completed by a sphincter‐like contraction of the outflow tract. Prior to maturation of the arterial valves, this area similarly prevents retrograde blood flow from the aortic arches. In addition to these functional differences, the cardiac segments can be distinguished by unique patterns of gene expression. While data suggest that the final determination of lineage fate likely occurs in the precardiac mesoderm [24, 55], the timing and nature of the mechanisms are incompletely understood. Perhaps the best‐studied molecular determinants of the dorsal–ventral axis in the gastrulating embryo are retinoids [56, 57]. Retinoids are products of vitamin A metabolism. Manipulation of retinoid signaling pathways results in significant abnormalities in axial patterning in general and cardiac development [58–60]. Abnormal development of the atrial segments and systemic venous tributaries is observed in conditions of retinoid deficiency [61–63]. Excess retinoids create malformations often involving the outflow tract [64, 65], and result in ventricular expression of several genes that are normally largely restricted to the atria at these stages of development [66–68]. The spatial and temporal patterns of retinoid signaling in early cardiac development are highly correlated with the presence of retinaldehyde dehydrogenase 2 (RALDH2), a key enzyme in the retinol (vitamin A) to retinoic acid conversion pathway [69–71]. Retinoid signaling pathways are clearly key mechanisms of segmental differentiation within the heart, but it is clear that other components intersect and augment this pathway. With the addition of cardiac tissue to the arterial and venous poles, the heart tube elongates and begins to bend. Looping becomes more complex as morphogenesis proceeds. The cranial portion of the tube bends caudally and to the right, while the caudal portion bends cranially to the left. The net outcome of this looping results in the left ventricle being positioned inferior and anterior to the atrium, while the right ventricle is slightly anterior and to the right of the left ventricle. The mechanisms regulating bending of the heart tube are incompletely understood. Interestingly, many looping movements are intrinsic to the heart, and can be observed even if the heart is isolated from the embryo, with or without beating [72, 73]. Evidence suggests that deformation of the linear heart tube into a looped structure may be a result of mechanical force [74]. Additionally, bending may simply be the result of asymmetric changes in cell shape. Alternatively, myocytes may replicate faster in the larger curvature of the loop, and more slowly in the lesser curvature [75, 76]. Additional studies suggest that asymmetric mechanical tension in the developing heart can induce bending. Experimentally, inhibitors of actin polymerization and cytoskeletal rearrangements can either abolish looping or reverse its direction, according to whether they have been universally or selectively applied [77]. Regardless of the regulatory mechanisms governing the process, bending of the heart tube confirms that the left and right sides of the embryo will not be morphologic mirror images. Although initial evidence of laterality is found in the atrioventricular canal prior to looping, cardiac development is inextricably linked to correct establishment of the three body axes [78, 79]. All vertebrate, and most invertebrate, body plans demonstrate fundamental asymmetry about the three body axes: cranial–caudal (C–C), dorsal–ventral (D–V), and left–right (L–R). At the molecular level, the axes are determined by asymmetric propagation of signaling events early in development. The process of L–R axis determination as it governs heart development is broadly conceptualized as requiring three steps, which involve the initiation, elaboration, and interpretation of the sidedness signals [80, 81]. The first step requires initiation of polarity along the C–C, D–V, and L–R axes. The second step is an elaboration and amplification of the initial L–R asymmetry. As is true in general for developmental processes, most of the molecules controlling the L–R signaling process in mice have recognized homologues in multiple species. Emphasizing the necessity to carefully examine multiple models, some of the molecules required for left‐sidedness in mice are determinants of right‐sidedness in birds [82]. The third step of axis determination is the interpretation of the asymmetric signals elaborated in the second step by the cells and tissues of developing organs. Paired structures develop as two fates, either as mirror symmetries, such as the limbs and parietal body parts, or as paired but unequal structures, such as the lungs, abdominal organs, and atrial appendages. These decisions are governed by both the signal delivered to the organ primordia as well as the reaction of the organ primordia to the signal. Such L–R axis determination in cardiac development is complex and several genetic models of abnormal cardiac looping have been described in mice [83]. Some models reflect an abnormal direction of looping, while others also show misalignment of cardiac segments. Mouse models of globally randomized arrangement of the organs [84, 85], global mirror imagery [86], defects affecting different embryonic organs [87], and defects with preferential bilateral right‐ or left‐sidedness resulting in isomerism have all been described [88]. Some genes implicated in the mouse models are likely also important in human isomerism [89]. There is often an increased incidence of abnormal ventriculo‐arterial connections in mouse models of abnormal L–R axis determination [73], suggesting an element of L–R signaling in normal development of the outflow tract. Looping determines not only the sidedness of the heart, but, importantly, the correct relationship of the segments of the heart to each other. After looping, it is possible to recognize inner and outer curvatures relative to the ventricular components of the heart tube (Figure 1.4). By nature of the tight angulation of the inner curvature, the primitive atrial, AV, ventricular, and outflow segments are near each other for the remainder of cardiac development. The inner curvature of the heart is arguably the most critical, complex, and dynamic site in normal cardiac development: it is the region where the right ventricle acquires its inflow, and the left ventricle acquires its outflow. On the luminal surface of the heart, the fold in the heart at the inner curvature results in a small muscular ridge inside the heart between the AV junction and the outflow tract called the ventriculo‐infundibular flange, or ridge. Other key landmarks are the two major endocardial cushions of the AV junction, and the two comparable endocardial cushions extending through the outflow tract. The inferior endocardial cushion is attached to the dorsal AV myocardium, while the superior cushion is attached to the ventral AV myocardium. Then, small right and left lateral AV endocardial cushions arise. The two outflow cushions in the outflow tract form extended spiral ridges, initially extending from the pericardial margins cranially to the body of the right ventricle caudally. The endocardial ridge ending in the anterior right ventricle is called the septal endocardial ridge. The endocardial ridge terminating posteriorly in the right ventricle is called the parietal endocardial ridge. The parietal endocardial ridge contacts the right lateral endocardial cushion, which itself will become continuous with the superior endocardial cushion. The septal endocardial ridge contacts the inferior endocardial cushion. As the atrioventricular endocardial cushions fuse during normal septation, together with the mesenchymal cap of the primary atrial septum and the dorsal mesenchymal protrusion, they create the central AV mesenchymal complex. This process continues, via the outflow cushions, to the distal extent of the myocardial outflow tract. The distal margin of the myocardial outflow tract, however, itself regresses proximally during development. The distal outflow tract is then separated by growth of an additional protrusion from the dorsal wall of the aortic sac. It is this protrusion that forms the embryonic aortopulmonary septum. At the most basic level, the process of fusion of mesenchymal tissues can be thought of as a tissue zipper that septates the heart [45]. With respect to human congenital heart disease, this is a critical series of events. Disease phenotypes such as transposition of the great arteries, common arterial trunk, and several tetralogy variants all derive from aberrations of these normal developmental steps. This developmental insight has informed the genetics of congenital heart disease. Similar, yet discrete, anatomic phenotypes may have mechanistically less to do with each other than more disparate anatomic phenotypes derived from common developmental mechanisms. Figure 1.4 The images are from an episcopic dataset prepared from a human embryo at Carnegie Stage 14, equivalent to day 11 of development in the mouse (E10.5). The left‐hand panel shows a section through the atrial and ventricular components of the developing heart after looping. The systemic venous sinus has been transferred to open to the right side of the atrial component of the primary heart tube, and the atrial appendages are beginning to balloon to right and left (white arrows with red borders). The primary atrial septum has begun to grow from the atrial roof toward the atrioventricular canal. The ventricular component of the heart tube has looped to the left, and the apical ventricular components are beginning to provide the primordiums of the developing right and left ventricles. The double‐headed white arrow shows the embryonic interventricular communication, which is roofed by the inner heart curvature, since at this stage the atrioventricular canal is supported exclusively by the developing left ventricle. The right‐hand panel shows how the developing right ventricle supports the outflow tract, which has proximal, intermediate, and distal components. Cushions extend through the intermediate and proximal components, but the distal part has a wide‐open lumen. Note that, at this early stage, the developing right atrium has no direct connection with the developing right ventricle, with the dashed white line showing the extensive right atrioventricular groove. AV, atrioventricular, iv, interventricular. The right AV junction is formed by rightward expansion of the AV junction, while at the same time the midline superior and inferior endocardial cushions are approaching each other in the center of the lumen of the AV junction. The outflow tract endocardial cushions, although unfused, define distinct proximal aortic and pulmonary channels. The aortic channel moves leftward and ventral of its original position. Because of the combined rightward expansion of the AV orifice, and the leftward movement of the aortic outflow tract, the acute angle of the inner curvature now defines the region where the AV junction and the outflow tract are in continuity. These same morphogenetic movements result in rotation of the proximal outflow cushions to a plane that is more closely parallel to that of the growing muscular ventricular septum. Following initial heart assembly, the various segments of the heart continue to develop simultaneously, yet often at different rates. For the rest of the chapter, these events will be presented from the standpoint of the final cardiac segments formed, rather than in the temporal order in which they develop. The embryonic systemic venous tributaries are formed by a process of vasculogenesis (Figures 1.5 and 1.6). Initially there are three bilaterally symmetric venous drainages: (i) the vitelline or omphalomesenteric, (ii) umbilical, and (iii) cardinal venous systems [90, 91]. The vitelline veins drain the embryonic gastrointestinal tract and gut derivatives. The umbilical veins bring oxygenated blood from the placenta to the heart. The cardinal venous system returns blood from the embryonic head, neck, and body wall. All three of these drainage systems enter the systemic venous component of the primitive heart tube, known as the sinus venosus. The final, adult pattern of venous drainage is established through complex patterns of regression, remodeling, and replacement of these embryonic venous systems and their connections to the definitive right atrium [92]. Figure 1.5 The images show how the systemic and pulmonary veins gain their connections to the right and left sides of the initial common atrial component of the primary heart tube. The left‐hand panel is a dorsal coronal section through the venous pole of the human embryo shown in Figure 1.1, at Carnegie Stage 14. The left superior caval vein is cut in its long axis as it extends through the dorsal left atrioventricular groove. It opens to the right side of the atrial components, along with the right‐sided superior caval vein and the inferior caval vein (SCV, ICV), with the boundaries of the systemic venous sinus marked by the venous valves. The section is cut through the connection of the atrial component of the heart tube to the pharyngeal mesenchyme, which is the dorsal mesocardium. The orifice of the pulmonary vein is visible in the center of the connection. The right‐hand panel is from an episcopic dataset prepared from a mouse embryo sacrificed at E11.5. It shows how the rightward margin of the dorsal mesocardium has proliferated to become the vestibular spine, with its growth serving to commit the pulmonary vein to the cavity of the developing left atrium. Note that the left superior caval vein (LSCV) has its own discrete walls as it runs behind the cavity of the left atrium to enter the atrioventricular groove. Initially, the systemic venous component is bilaterally symmetric, with no anatomic boundaries initially present between it and the atrial component of the heart tube. As development progresses, the junction of tributaries and atrium shifts toward the right, perhaps influenced from blood shunting left to right. Normally, the connections of the left‐sided cardinal, vitelline, and umbilical venous systems with the left horn of the systemic venous component regress. This results in the coronary sinus remaining as the primary structural derivative of the left horn of the systemic venous sinus in the normal fetal and postnatal heart. Failure of regression of the connections with the left venous horn results in persistence of the left superior caval vein. The right horn of the systemic venous sinus normally accommodates the entirety of the systemic venous drainage, except the portion from the heart, which is returned via the coronary sinus. A portion of this right horn is incorporated into the right atrium to form its smooth systemic venous portion between the orifices of the caval veins. At the same time, it is possible to recognize valves at the boundaries between the atrial and venous components. The inferior portion of the rightward venous valves becomes the Eustachian and Thebesian valves. They are related to the orifices of the inferior caval vein and the coronary sinus, respectively. During these processes, the umbilical and vitelline plexuses remodel and regress. Remodeling of the umbilical system produces the venous duct, or ductus venosus, which retains its connection with the cardinal system so as to connect to the developing inferior caval vein, thus bypassing liver sinusoids derived from the vitelline system. After birth, the venous duct becomes ligamentous. Hence, no derivatives of the embryonic umbilical venous drainage connect to the heart or persist in postnatal life. The cardinal venous system initially consists of bilateral anterior cardinal veins, which drain the cephalic portion of the embryo, and bilateral posterior cardinal veins, which drain the caudal portion of the embryo. Fusion of the cardinal veins at the level of the systemic venous sinus forms the sinus horns, or common cardinal veins. The left anterior cardinal vein eventually loses its connection with the left sinus horn, but a small remnant on the surface of the heart normally persists as a passage of coronary venous blood to the coronary sinus, and is known as the oblique vein of the left atrium. Another portion of the left anterior cardinal vein persists as the left internal jugular vein. As the left anterior cardinal vein loses connection with the heart, it becomes connected to the right anterior cardinal vein via the intercardinal anastomosis that forms between the thyroid vein and the thymic vein. This connection persists as the left brachiocephalic vein. The portion of the right anterior cardinal vein between the right atrium and the drainage of the left anterior cardinal vein proximally, via the intercardinal anastomosis, becomes the normal right superior caval vein. Figure 1.6 The images are from a serially sectioned human embryo at Carnegie Stage 14. The upper section has been incubated with an antibody to NKX 2.5, which marks the myocardium of the atrial walls and the proximal part of the outflow tract. The opening of the pulmonary vein can be seen between the margins of the dorsal mesocardium. The antibody does not, however, mark the walls of the systemic venous tributaries. As is shown in the lower panel, which is an adjacent serial section but incubated with an antibody to TBX18, the venous myocardium is positive to the second antibody, which does not mark the myocardium of either the atrial walls or the outflow tract. The findings support the notion that the pulmonary venous myocardium is not derived from the systemic venous component of the developing heart. Reproduced with the permission of Aleksandr Sizarov. The posterior cardinal veins originally drain the body wall, gonadal, and renal structures. Their function in venous drainage of the body wall is supplanted by the supracardinal venous plexus. The gonadal and renal venous drainage is captured by the subcardinal venous plexus and the lower extremities are drained by the sacrocardinal veins. The various venous beds contribute the segments that form the definitive inferior caval vein to the level of the persisting segment derived from the right vitelline vein, which then connects to the right atrium. The supracardinal venous system persists as the azygous and hemiazygous veins. In both the supracardinal and subcardinal venous systems, the initial vascular structures are bilaterally symmetric. The left‐sided channels subsequently regress, underscoring the typical right‐sided location of the inferior caval vein. The frequency of venous drainage abnormalities in human and animal models of altered L–R axis differentiation [88] strongly suggest that the mechanisms of venous morphogenesis depend upon appropriate left–right signaling. The earliest evidence of the formation of the common pulmonary vein in the embryo is the presence of a strand of endothelial cells in the pharyngeal mesenchyme, pointing to the dorsal mesocardium. This endothelial strand forms a lumen, and initially is a midline structure. As the vestibular spine is developing and projecting into the atrial cavity on the right side of the primitive pulmonary vein, the relative position of the common pulmonary vein changes as it occupies a position to the left of center [93]. The continued development of the vestibular spine [44], the myocardialization of this mesenchyme, and the concomitant growth of the primary atrial septum eventually result in the normal connection of the pulmonary vein to the left atrium [94]. The development of the pulmonary vein does not result from an outgrowth of the atrial wall. During this process, the lungs themselves develop from the foregut, with venous elements from the lungs bilaterally fusing with the common pulmonary vein. Abnormal formation of the common pulmonary vein results in persistence of initial connections of the pulmonary plexuses to the cardinal veins, resulting in various forms of anomalous pulmonary venous return. Initially, the walls of the pulmonary veins are not muscular. As development proceeds, myocardial sleeves are formed around these veins [95]. Semaphorin signaling has been linked to defects in this process [96]. As pulmonary myocardium is a frequent site initiating atrial fibrillation, a clear understanding of the molecular and genetic mechanisms that direct these events is important. The major steps in cardiac septation occur between the fourth and fifth weeks of human gestation. Proper atrial septation requires two important processes. The first is differential proliferation of tissues: for example, the folds often described as the “septum secundum.” The second process is active proliferation of cells that approach each other and fuse, resulting in septation of the chamber: for example, growth of the primary septum and the endocardial cushions. Atrial septation and connection of the common pulmonary vein to the left atrium are closely related events in the normal heart [94]. Experiments in mouse, chick, and human embryos highlight the importance of the dorsal mesocardium to these events [44]. Unlike other mesenchymal tissues in the AV junction, the vestibular spine, which grows through the rightward margin of the dorsal mesocardium, is not derived from an epithelial‐to‐mesenchymal transformation, but rather is a derivative of the second heart field [28, 45]. The vestibular spine extends into the atrial cavity, becoming contiguous with the mesenchymal cap on the leading edge of the primary atrial septum. Together with the superior and inferior atrioventricular cushions, the vestibular spine and the cap eventually fuse to form the atrioventricular mesenchymal complex. This process is essential for normal atrioventricular septation (Figure 1.6). Recent work suggests that perturbation of the development of the protrusion might be one of the contributing mechanisms in the pathogenesis of atrioventricular septal defects [97].
CHAPTER 1
Development of the Heart and Great Vessels
Origin of Cardiac Precursor Cells
Human
Mouse
Chicken
Carnegie Stage
Streeter horizon
Days’ gestation
Embryonic days (Theiler’s stage)
Hamburger/Hamilton stage (days of incubation)
Human cardiac developmental milestone
9
IX
20
8–8.5 (12)
7–8 (1.1)
Circulation begins
10
X
22
8.5–9 (13)
10 (1.5)
11
XI
24
9–9.5 (14)
11 (1.8)
12
XII
26
9.5–10.25 (15)
14 (2.2)
13
XIII
28
10.25–10.5 (16)
17 (2.6)
Looping
14
XIV–XV
32
10.5–10.75 (17)
19 (2.9)
Atrial septation begins
15
XVI
33
11 (18)
20–21 (3.3)
OFT septation begins
16
XVIII
37
11.5 (19)
24 (4)
Ventricular and AV septation begins
17
XX
41
12 (20)
26 (4.8)
18
XXI–XXII
44
12.5 (21)
28 (5.6)
Valvar maturation
19
XXIII
47
13 (21)
29–30 (6.4)
20
50
14 (22)
31–32 (7.2)
21
52
14 (22)
34 (8)
22
54
14 (22)
35 (8.7)
23
56
14 (22)
36 (9.6)
Formation of the Heart Tube and Early Segmentation
Looping
Development of the Inflow (Venous) Pole of the Heart
Systemic Venous Inflow
Pulmonary Venous Inflow
Atrial Septation
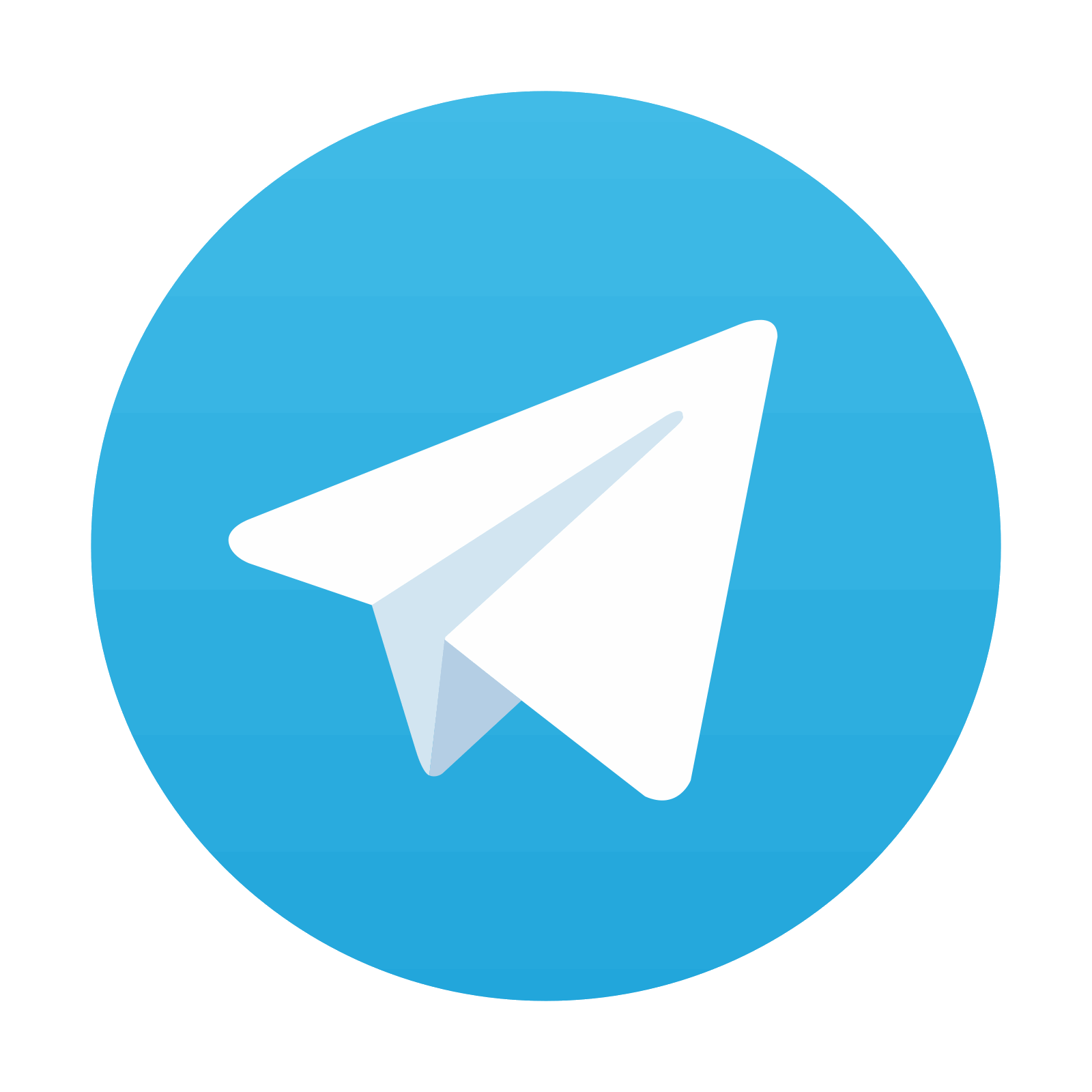
Stay updated, free articles. Join our Telegram channel
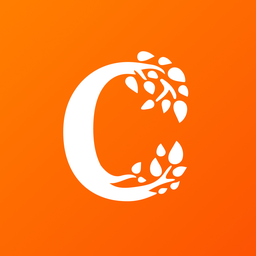
Full access? Get Clinical Tree
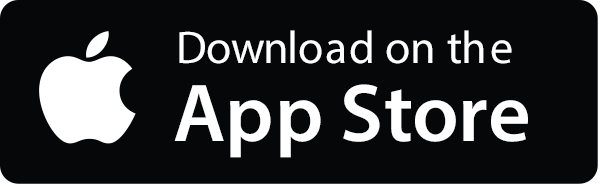
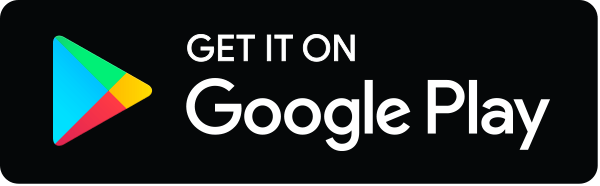