The physical signs of cyanosis and decreased oxygen saturation are usually the result of hypoxemia, and can be found in newborn infants with a variety of pathologies. Hypoxemia can be the result of shunting of deoxygenated blood from the venous to the arterial circulation. This can occur at many levels including intracardiac, extracardiac, and intrapulmonary locations. The primary causes of hypoxemia in infants without congenital heart disease vary and include pulmonary problems such as pneumonia, respiratory distress syndrome (RDS), congenital anomalies, pneumothorax, and meconium aspiration syndrome (MAS). Additionally, the primary cause of hypoxemia could be persistent pulmonary hypertension of the newborn (PPHN), a failure or delay in making the circulatory transition from fetal to newborn life leading to persistently elevated pulmonary vascular resistance, and continued shunting through the fetal channels. In most cases, PPHN is secondary to another respiratory disease, and therefore these findings do not usually occur in isolation. Certain conditions predispose infants to having a more prolonged circulatory transition. Infants at higher risk include infants of diabetic mothers, infants with trisomy 21, and fetal exposure to nonsteroidal anti-inflammatory agents or selective serotonin reuptake inhibitors. Preterm infants may have a variety of transitional problems after birth leading to hypoxemia. Both RDS and prolonged premature rupture of membranes (PPROM) are associated with PPHN. Additionally, a significant patent ductus arteriosus (PDA) is often associated with increasing oxygen and ventilator requirements. Echocardiography can be helpful in the diagnosis and management of many of these problems.
To differentiate the causes of hypoxemia, the neonatologist should perform and interpret a complete, comprehensive echocardiographic study to exclude congenital heart disease. When interpreting the echocardiogram, one must consider the clinical circumstances associated with the presentation. The neonatologist is in a unique position to be thoroughly involved with the clinical care of the infant and can determine whether the clinical course and the echocardiography findings are congruent. When the clinical course and the echocardiography findings are inconsistent, it is important for the neonatogolist to further evaluate all of the findings. The neonatologist performing echocardiography should readily refer to a pediatric cardiologist whenever congenital heart disease is suspected. In recent years, routine oxygen saturation screening of the newborn prior to discharge from the hospital has been recommended to detect otherwise silent critical congenital heart disease that could cause serious complications if left undetected.1,2 These screens have frequently identified infants with hypoxemia not caused by critical congenital heart disease. Approximately one-third of the infants with a positive screen result that is not associated with critical congenital heart disease will have another illness requiring treatment.3,4
In addition to assessment of the immediate transitional problems that cause hypoxemia, echocardiography can be helpful in evaluating infants at any time during the neonatal intensive care unit course when clinical deterioration occurs. Infants on the ventilator may have impaired cardiac function because of excessive intrathoracic pressure. Pleural and pericardial effusions can develop as complications from invasive central lines and are easily identified on the ultrasound examination. Infants with bronchopulmonary dysplasia or sepsis can have hemodynamic compromise that can be evaluated by echocardiography. Thus, echocardiography should be considered an important tool to assist in the intensive care management of any newborn infant. Many treatment strategies for neonatal diseases vary by institution and geographic location. Particularly in the case of PPHN treatment, strategies have been documented to be quite variable.5 Using echocardiography to guide therapy is a useful approach to individualizing treatment. However, many challenges in consistently accomplishing such an approach continue to exist, including the lack of evidence for specific treatments in relation to echocardiographic findings.
In this chapter, the constellation of echocardiographic findings in a variety of disease states will be reviewed. Whenever available, currently existing evidence for echocardiography-guided treatment will be discussed. The echocardiographic findings for each standard view that might be seen in infants with hypoxemia will be reviewed. The specific findings of congenital heart disease will not be discussed except for a few key features that should trigger a more thorough anatomic evaluation. Echocardiographic findings that can be used to influence management will also be presented.
Since the establishment of echocardiography as a superior, noninvasive, and readily available diagnostic modality, investigators have evaluated a variety of methods that can utilize this technique to help differentiate the causes of hypoxemia and predict the outcome and response to therapy. Decades ago, Johnson et al6 evaluated 16 infants with primary pulmonary disease (pneumonia, MAS, and RDS) requiring 100% oxygen. Systolic time intervals, right ventricular pre-ejection period/right ventricular ejection time ratio (RPEP/RVET), and left ventricular pre-ejection period/left ventricular ejection time ratio (LPEP/LVET) were compared among infants who responded to tolazoline and those who did not. Half of the infants responded to tolazoline; this cohort had prolonged RPEP/RVET and LPEP/LVET ratios compared with those who did not. This suggests that infants with a responsive pulmonary vasculature such as in PPHN are more likely to have prolonged ventricular systolic time intervals. Walther et al7 compared 33 infants treated with high oxygen concentrations and mechanical ventilation in the first day of life with 32 control infants. Half of the infants with high oxygen requirements met criteria for extracorporeal membrane oxygenation (ECMO). Compared with controls, those with high oxygen requirements had lower aortopulmonary pressure differences, larger diameter and more prolonged patency of the ductus arteriosus, and more right-to-left ductal shunting. Infants meeting ECMO criteria had very low aortopulmonary pressure differences. Similarly, Evans et al8 used serial echocardiography to evaluate 32 term infants requiring more than 70% oxygen. Echocardiograms were performed at presentation, and then daily until the oxygen requirement was less than 30%. Infants were divided into a primary respiratory pathology group and a primary PPHN group. Those with primary PPHN had a higher incidence of PDA and larger PDAs. Similar numbers of infants in each group had bidirectional or pure right-to-left shunting at the PDA. Both groups had a frequent occurrence of low ventricular output (both right and left ventricles), which increased with the clinical improvement of the infants. Overall, in both groups only approximately 50% of the infants had suprasystemic pulmonary artery pressures, and pulmonary artery pressure decreased before the hypoxemia improved. The authors concluded that most of the hypoxemia in these infants was caused by intrapulmonary right-to-left shunting rather than shunting through the PDA and patent foramen ovale (PFO).
These studies show that the constellation of echocardiographic findings in infants with high oxygen requirements frequently includes abnormal systolic time intervals (a sign of increased afterload), prolonged patency of the ductus arteriosus, smaller aortopulmonary pressure differences with more right-to-left shunting through the PDA (a sign of higher pulmonary artery pressures), and decreased ventricular outputs.
Many studies have shown that infants with RDS have delayed transitional circulation with evidence of higher pulmonary artery pressure and lower aortopulmonary pressure differences in the first several days after birth. In 1977, Halliday et al9 published an echocardiographic evaluation of infants with RDS over the first 5 days of life finding prolonged RPEP:RVET in infants with more severe clinical disease. Randala et al10 found that preterm infants with RDS treated with surfactant had a lower systemic to pulmonary artery pressure difference than preterm infants without RDS and term infants at 48 and 72 hours of age, yet all groups were similar at 24 hours of age. Evans and Archer11 found that preterm infants with RDS had signs of increased pulmonary artery pressure estimated by right ventricular systolic time intervals compared with infants without respiratory distress. The systolic time intervals did not correlate well with clinical disease but did correlate with the level of bidirectional shunting at the PDA and PFO. These authors also showed that the ductus arteriosus closed more slowly in infants with RDS. Bidirectional PDA flow occurred in 40% of infants with RDS at 19 hours of life, but most had become completely left to right by 60-79 hours of life. In preterm infants, Seppanen et al12 showed that those with RDS more frequently had tricuspid regurgitation (TR) than healthy preterm infants, and pulmonary artery pressure decreased more slowly in infants with RDS. Evans and Kluckow13 documented a higher incidence of low right ventricular output (<150 ml/kg/min) in preterm infants with more severe RDS. Taken together, these studies show evidence of relatively elevated pulmonary arterial pressure and prolonged ductal patency in infants with RDS.
It is well known that poor glycemic control during pregnancy is associated with hypertrophic cardiomyopathy.14,15 The echocardiogram will show an increased interventricular septum thickness. The ventricular free wall thickness may also be increased but often not as much as the septal wall (asymmetric septal hypertrophy). Functional ventricular outflow obstruction can be seen with decreased left ventricular output.16 Infants of diabetic mothers are also at risk for delayed circulatory transition with increased pulmonary artery pressure and prolonged persistence of the ductus arteriosus.17,18 Even when glucose levels are tightly managed during pregnancy, infants show signs of delayed circulatory transition with lower right ventricular output than control infants.19
Another condition that can be associated with hypertrophic cardiomyopathy is fetal closure of the ductus arteriosus. Gewilling et al20 reviewed the echocardiographic findings in 12 cases of fetal ductus arteriosus closure. Important abnormalities included right ventricular hypertrophy, tricuspid and pulmonary regurgitation, and right atrial dilation. Such findings demonstrate the consequences of prolonged exposure to excessive afterload during fetal life on the right ventricle. These patients are at increased risk of hypoxemia due to right-to-left shunting at the fetal channels secondary to pulmonary hypertension.
Fewer echocardiographic studies have focused on the findings associated with MAS, even though this is one of the more common diseases leading to PPHN. However, in one study, Korhonen et al21 compared serial echocardiography findings in 17 infants with mild to moderate MAS with those in 16 healthy matched control infants over the first 3 days of life. They found more frequent bidirectional shunting at the ductus arteriosus at 12 hours of life in infants with MAS. Additionally, infants with MAS had a lower aortopulmonary pressure gradient over the first 12 hours of life. In this population of infants, none had pure right-to-left ductal shunting and the left ventricular output was not compromised. On the other hand, infants with MAS had a lower-velocity time integral in the left pulmonary artery. Therefore, hypoxemia in the setting of MAS is multifactorial, which includes low pulmonary flow, right-to-left shunting at the fetal channels, and intrapulmonary V:Q mismatch.
Oxygen is often recognized as a pulmonary vasodilator, although in excess can blunt vasodilation response to nitric oxide. To determine the hemodynamic response to different levels of oxygenation, Skinner et al22 performed echocardiograms on 18 preterm infants with respiratory failure at three levels of arterial oxygen saturation (SpO2): 86%, 96%, and 100%. The level of oxygenation was adjusted in each infant by changing FiO2 and maintaining the SpO2 at the desired level for 15 minutes. Infants with lower SpO2 had more signs of elevated pulmonary artery pressure as evidence by lower aortopulmonary pressure differences from Doppler assessment of the PDA flow. Higher oxygen saturation levels were also associated with greater constriction of the ductus arteriosus. In contrast, in a group of 13 preterm infants, Bard et al23 found no differences in pulmonary artery pressure measured by velocity of the TR jet between SpO2 levels of 95% versus 90%. The differences in findings between these studies may be related to the sensitivity of the marker evaluated in each study or to the differences in oxygen saturation levels.
Assisted ventilation has the potential to alter neonatal hemodynamics in several ways. Increasing intrathoracic pressure may increase pulmonary vascular resistance, decrease pulmonary blood flow, and decrease systemic venous return. Alternatively, optimizing lung volume could decrease pulmonary vascular resistance and increase pulmonary blood flow. The actual effects of changes in intrathoracic pressure from assisted ventilation can most depend upon the level of illness of the lungs. De Waal et al24 evaluated the hemodynamic effects of altering the positive end expiratory pressure (PEEP) level between 5 and 8 cm H2O in 50 ventilated infants. Increasing PEEP to 8 cm H2O was associated with a significant change in superior vena cava (SVC) flow in 36% of infants, but the direction of change increased in half of those and decreased in the other half. Improving pulmonary compliance tended to improve systemic blood flow measures. The right ventricular output was decreased on average with increasing PEEP. One of the risk factors for low SVC flow in preterm infants is higher mean airway pressure.25 Osborn and Evans26 performed a randomized controlled trial comparing the hemodynamic effects of high-frequency oscillatory ventilation (HFOV) with conventional ventilation. There were no statistically significant differences found in the SVC flow or right ventricular output. However, a nonsignificant trend in terms of increased incidence of low SVC flow was found with HFOV, and more infants in the HFOV group were treated with dobutamine.
The hemodynamic response to surfactant dosing remains incompletely understood. Hamdan and Shaw27 evaluated the ratio of pulmonary artery acceleration time (PAAT) to RVET (PAAT:RVET) in infants immediately before each of 2 doses of exosurf were given to a group of preterm infants. They then repeated measures 1 and 6 hours after each dose and 12, 36, and 60 hours after the second dose. They found that pulmonary artery acceleration time to right ventricular ejection time (PAAT:RVET) steadily increased over this time period but the rate of increase was higher at 1 hour after each surfactant dose. Kaapa et al28 found a decrease in pulmonary artery pressure and an increase in left-to-right PDA flow after surfactant dosing as compared to infants undergoing endotracheal suctioning who did not have these changes. By contrast, Bloom et al29 evaluated 12 preterm infants with severe RDS treated with natural surfactant and 25 preterm infants with mild RDS. They found significantly lower aortopulmonary pressure difference in infants with severe RDS compared with those with mild RDS. These aortopulmonary pressure differences did not increase immediately after surfactant administration but became similar to infants with mild RDS by 48 hours of age. They also found lower levels of left pulmonary artery blood flow in infants with more severe RDS. More recently, in a study of delivery room surfactant administration to preterm infants less than 30 weeks’ gestation, Sehgal et al30 found that PDA shunting became all left to right after surfactant dosing given within 30 minutes of birth. In summary, these studies show that infants with RDS have signs of increased pulmonary artery pressure compared with infants who do not have RDS. Surfactant therapy is associated with a reduction in pulmonary artery pressure at varying time frames after dosing.
Each clinician must have an organized and standardized approach to performing an echocardiogram. This same approach should be followed with each examination, so that no images/views are missed. Similarly, clinicians should follow a standardized approach when reviewing the images to ensure that all aspects were assessed. The following is one approach that can be used. Individual clinicians may choose a different order of imaging as long as all areas are addressed. The discussion of each of the following images focuses on the findings associated with diseases causing hypoxemia. A set of images taken from newborn infants with a variety of clinical conditions will follow each description. In interpreting the echocardiographic images, it is critical for the clinician to remember that the findings are very dynamic over the first several days of life. Therefore, when evaluating the sick infant, one must consider the normal changes that can occur during that same time frame. In the first 3 days of life, knowledge of the age of the patient is very important for the interpretation of the echocardiogram.
In the parasternal long-axis view, it is important to evaluate the relative size of the chambers and the function of the ventricles. In PPHN, pulmonary blood flow is low and therefore pulmonary venous return is decreased and the left atrium and left ventricle can appear underfilled. An interventricular septum that is flattened or bulging into the left side can often be seen in the long-axis view. Myocardial hypertrophy can also be evaluated from this view. Measures of systolic function such as shortening fraction can be obtained using an M-mode tracing through the interventricular septum at the level of the mitral valve (see Chapters 5 and 8). It should be remembered that these measures are not reliable when the ventricular motion is not uniform or the shape is distorted. Decreased left ventricular systolic function is not a frequent finding in PPHN. The relative size of the left atrium and aortic root can also be measured in this view. The aortic valve diameter should be measured in this view for calculation of the left ventricular output. In infants with impairment of myocardial systolic function, mitral insufficiency can often be identified in this view using color flow Doppler.
Figure and Video 13-1 is an example of a long-axis view from a 30-week preterm infant with mild RDS at 4 hours of life. This image shows normal cardiac anatomy (from this perspective) and function. Figure and Video 13-2 is an example of this view in a 25-week preterm infant with a large mostly left-to-right PDA at approximately 4 hours of life. Figure and Video 13-3 shows the same infant at 20 hours of life with a closed PDA after 1 dose of indomethacin. Notice the difference between the two clips in the size of left atrium. Figure and Video 13-4 shows this view from an infant with prolonged rupture of membranes at 2 hours of life. Note the somewhat thickened interventricular septum and the small left atrium. Figure and Video 13-5 shows the long-axis view from a term infant who failed the oxygen saturation screen and was thought to have fetal closure of the ductus arteriosus. Note the hypertrophied right ventricle. Figure and Video 13-6 shows the long-axis view from an infant with severe oligohydramnios associated with renal anomalies at 3 hours of life. Figure and Video 13-7 shows the color Doppler imaging from this view in the same infant. Note the mitral regurgitation that is present at this time. In Figure and Video 13-8 we see the long-axis view from this infant at 13 hours of life at a time when the infant developed higher oxygen requirements and increasing acidosis. Note the visibly poor function in this clip with the infant on HFOV. Figure and Video 13-9 shows this infant on a brief trial of conventional mechanical ventilation. Finally, in Figure and Video 13-10 we see imaging from the same infant after 7 hours on a continuous epinephrine infusion. This series of clips demonstrates a range of findings that can be observed in this single view even in an individual infant followed over time as the disease progresses.
FIGURE and VIDEO 13-3.
The same infant as in Figure and Video 13-2 at 20 hours of life with a closed ductus arteriosus after 1 dose of indomethacin. Notice the difference between the two videos in the size of left atrium.

FIGURE and VIDEO 13-7.
A color Doppler imaging of the long-axis view of same patient as in Figure and Video 13-6. Note the mitral regurgitation that is present at this time.

FIGURE and VIDEO 13-8.
A long-axis view from the same patient as in Figures and Videos 13-6 and 13-7 at 13 hours of life at a time when the infant developed higher oxygen requirements and increasing acidosis. Note the visibly poor function in this clip with the infant on high-frequency ventilation.

FIGURE and VIDEO 13-10.
A long-axis view from the same patient as in Figures and Videos 13-6 to 13-9 after 7 hours and on an epinephrine drip.

With color Doppler, TR can be identified and with CW Doppler the velocity of the TR jet can be measured. With the modified Bernoulli equation, the pressure difference between the right ventricle and right atrium can be calculated and the pulmonary artery pressure can be estimated (see Chapter 12). Figure and Video 13-11 shows the right ventricular inflow view using color Doppler of a 30-week preterm infant with mild RDS at 4 hours of age. Figure and Video 13-12 shows the right ventricular inflow view from an infant with PPROM at 2 hours of life. Figure and Video 13-13 shows this view from an infant with fetal closure of the ductus arteriosus. Note the tricuspid regurgitation (TR) in Figures and Videos 13-12 and 13-13. Figures and Videos 13-14 and 13-15 show the two-dimensional (2D) and color Doppler imaging of the right ventricular inflow view in an infant with severe oligohydramnios. Figure 13-16 shows the CW Doppler trace from an infant with significant TR.
When the pulmonary valve is visualized, the leaflets should be seen opening and closing. With color Doppler the blood flow out of the right ventricle can be evaluated. Normal flow should be laminar, and this is seen as a uniform color hue. Turbulent flow suggests a physical obstruction to flow. A hypertrophic ventricle can lead to ventricular outflow tract obstruction leading to turbulent flow with increased velocity seen distal to the obstruction. A pulsed wave (PW) Doppler tracing is obtained with the range gate placed just distal to the pulmonary valve. The shape of the waveform can give an indication of the presence of pulmonary hypertension with a rapid acceleration limb and notching on the deceleration limb. The RPEP:RVET and time to peak velocity (TPV) to RVET ratio (TPV:RVET) can be measured as previously described (Chapter 12), and if abnormal, these measures give an indication of the presence of increased afterload. If a PDA with left-to-right shunting is present, the color Doppler signal from the PDA can be seen coming into the main pulmonary artery and can cause a signal of continuous systolic/diastolic flow into the main and branch pulmonary arteries by PW and continuous-wave (CW) Doppler.31 The right ventricular output will be measured in this view using the diameter of the pulmonary valve and the velocity time integral and heart rate from the PW Doppler tracing.
Figure and Video 13-17 shows the right ventricular outflow view from a 30-week preterm infant with mild RDS at 4 hours of life. Figure 13-18 show the PW Doppler trace in the main pulmonary artery in this infant. The diastolic flow reflects PDA left-to-right shunting into the pulmonary artery. Figure and Video 13-19 shows the right ventricular outflow view from the recipient infant of TTTS. Note the more hypertrophied appearance of the RV. Figure and Video 13-20 shows the pulmonary artery from an infant with PPROM. Figures and Videos 13-21 and 13-22 show the 2D and color flow Doppler imaging of the right ventricular outflow from an infant with fetal closure of the ductus arteriosus. Figure 13-23 shows the PW Doppler in the main pulmonary artery of this infant demonstrating a notching pattern with a rapid acceleration limb of the tracing, signifying elevated pulmonary artery pressure (pulmonary hypertension). Figures and Videos 13-24 and 13-25 show the 2D and color flow Doppler imaging from an infant with severe oligohydramnios at 3 hours of life. Compare these with Figure and Video 13-26 taken from this infant at 13 hours of life and Figure and Video 13-27 once the infant had been treated with a continuous epinephrine infusion. Figures 13-28 and 13-29 show the PW Doppler tracing from this infant at 13 hours of life when the function was at its worst, and at 20 hours after treatment with epinephrine.
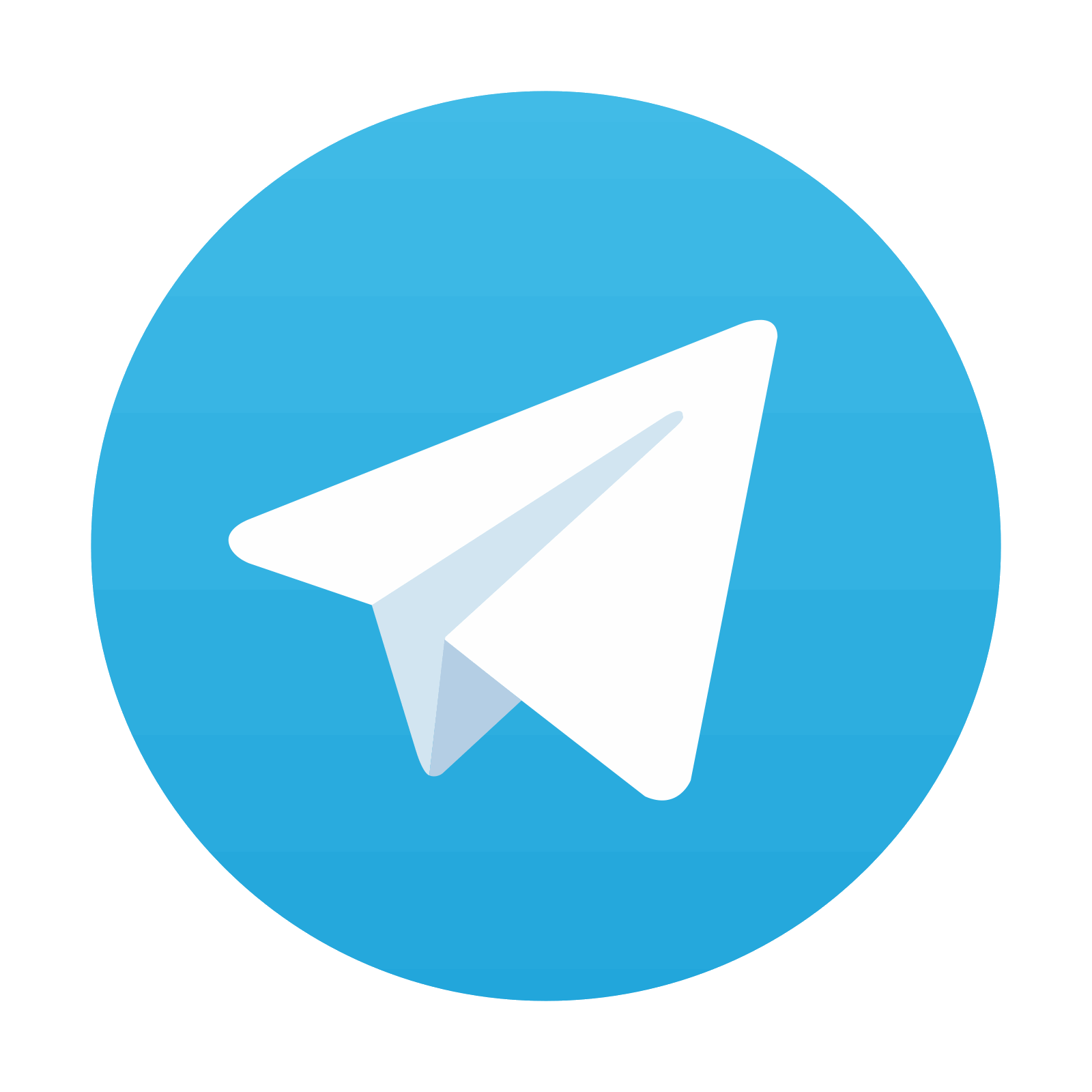
Stay updated, free articles. Join our Telegram channel
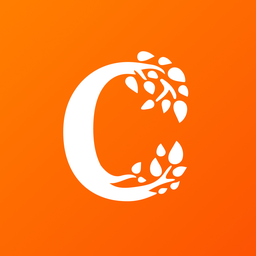
Full access? Get Clinical Tree
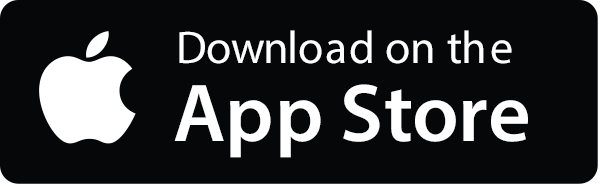
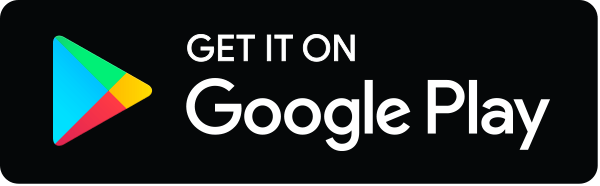