Objectives
- 1.
Provide an overview of the three basic elements of the ventilatory control system.
- 2.
Explain the structure and function of central chemoreceptors and peripheral chemoreceptors and their interrelationship.
- 3.
Describe five chest wall and lung reflexes important in the control of respiration.
- 4.
Describe the anatomy of the central respiratory control center and the relationship between the ventral and dorsal respiratory groups.
- 5.
Describe the role of cerebrospinal fluid hydrogen ion and HCO 3 – in the regulation of respiration.
- 6.
Explain the effects of hypoxemia, increased work of breathing, sleep, and acidosis on the ventilatory response to CO 2 .
- 7.
List three diseases associated with abnormal respiratory control.
Respiration demonstrates automaticity as well as self-modulation (voluntary). Although intermittent respiratory movements have been observed in utero, regular, automatic respiration begins at birth. Inspiration and exhalation occur automatically under the control of neurons located in the brainstem. At the same time, however, voluntary hyperventilation is easy, breath-holding is possible within limits, and the breathing pattern is modulated by the need for speech and singing.
Ventilatory control refers to the generation and regulation of rhythmic breathing by the respiratory center in the brainstem and its modification by the input of information from higher brain centers and systemic receptors. From a mechanical perspective, the goal of breathing is to minimize work; from a physiologic perspective, the goal is to maintain blood gas levels and specifically to regulate arterial P co 2 . A third goal of breathing is to maintain the acid–base environment of the brain through the effects of ventilation on arterial P co 2 .
Overview of Ventilatory Control
From a functional perspective, the ventilatory control system consists of three basic elements ( Fig. 10.1 ):
- 1.
Sensors (peripheral and central chemoreceptors and pulmonary mechanoreceptors) that gather information and feed that information to the central controller.
- 2.
The central controller (the respiratory control center) located in the brain that integrates and coordinates the information and sends signals to the effectors.
- 3.
The effectors (respiratory muscles including the diaphragm) that produce changes in the ventilatory pattern.

As described in Chapter 5 , alveolar ventilation is a function of respiratory rate and tidal volume. Respiratory rate is determined by the signal frequency from the central controller to the effectors, whereas tidal volume is determined by the activity of the individual nerve fibers in the effectors to their motor units, including the frequency and duration of discharges and the number of units activated.
The respiratory control center is located in the reticular formation of the medulla oblongata beneath the floor of the fourth ventricle. This center is not a discrete nucleus, but rather a poorly defined collection of different nuclei that generate and modify the basic rhythmic ventilatory pattern. It consists of two main parts: a ventilatory pattern generator , where the rhythmic pattern is generated; and an integrator , which processes inputs from higher brain centers and chemoreceptors and controls the rate and amplitude of the ventilatory pattern. The integrator controls the pattern generator and determines the appropriate ventilatory drive. Input to the integrator arises from higher brain centers including the cerebral cortex, hypothalamus, amygdala, limbic system, and cerebellum.
Within the central nervous system, central chemoreceptors are located just below the ventrolateral surface of the medulla. These chemoreceptors detect changes in the P co 2 /pH of brainstem interstitial fluid and modulate ventilation.
Peripheral structures also provide input to the integrator and control ventilatory drive. Chemosensitive peripheral chemoreceptors are located on specialized cells in the aortic arch ( aortic bodies ) and at the bifurcation of the internal and external carotid arteries in the neck ( carotid bodies ). These chemoreceptors respond to changes in their local environment associated with decreases in P o 2 , increases in P co 2 , and decreases in the pH of arterial blood and give afferent information to the central respiratory control center through the vagus nerve (aortic bodies) and the carotid sinus nerve , a branch of the glossopharyngeal nerve (carotid bodies), to make adjustments in alveolar ventilation that change whole body P co 2 , pH, and P o 2 . Finally, the ventilatory pattern can be modulated by pulmonary mechanoreceptors and irritant receptors in the lung in response to the degree of lung inflation or the presence of an irritant in the airways.
The collective output of the respiratory control center to the motor neurons controls the muscles of respiration (the effectors), and it is this output that results in automatic, rhythmic respiration. The responsible motor neurons are located in the anterior horn of the spinal column. Intercostal muscles and the accessory muscles of respiration are controlled by motor neurons located in the thoracic region of the spinal column. Diaphragmatic motor neurons are situated in the cervical region of the spine and control diaphragmatic activity through the phrenic nerve.
In contrast to automatic respiration, voluntary respiration bypasses the medullary respiratory control center. It originates in the motor cortex, with information passing directly to the motor neurons in the spine through the corticospinal tracts. The respiratory muscle motor neurons thus act as the final site of integration of voluntary (corticospinal tract) and automatic (ventrolateral tract) control of ventilation. Voluntary control of these muscles competes with automatic influences at the level of the spinal motor neurons and can be demonstrated by breath-holding. At the start of a breath hold, voluntary control dominates the spinal motor neurons, but as the breath hold continues, automatic ventilatory control eventually overpowers the voluntary effort and limits the duration of the breath hold.
There are also motor neurons that innervate the muscles of the upper airway. These are located within the medulla near the respiratory control center. They innervate muscles in the upper airways through cranial nerves. When activated, they result in dilation of the pharynx and large airways at the initiation of inspiration.
The Respiratory Control Center
Most of what is known about the control of ventilation comes from studies in animals in which focal areas of the brain have been destroyed (ablation) or in which the brainstem has been surgically cross-cut. When the brain is cross-cut between the medulla and the pons, periodic breathing is maintained even if all other afferents to the area, including the vagus nerve, are severed, demonstrating that the inherent rhythmicity of breathing originates in the medulla. If the brain is transected below this area, breathing ceases. Although no single group of neurons in the medulla has been found to be the breathing “pacemaker,” there are two distinct groups of nuclei within the medulla that are involved in respiratory pattern generation ( Fig. 10.2 ).

The first group is the dorsal respiratory group (DRG), which is composed of cells in the nucleus tractus solitarius located in the dorsomedial region of the medulla. These cells have the property of intrinsic periodic firing and are primarily responsible for inspiration and for the basic rhythm of ventilation. The tractus solitarius is also the primary projection site of visceral afferent fibers of the 9th cranial nerve (glossopharyngeal) and the 10th cranial nerve (vagus). These nerves provide information about P o 2 , P co 2 , and pH from peripheral chemoreceptors and systemic arterial blood pressure. The vagus nerve also carries information from pulmonary mechanoreceptors. The input from the 9th and 10th cranial nerves to the DRG is thought to constitute the initial intracranial processing station for these afferent inputs. However, even when all afferent stimuli to the DRG have been abolished, the DRG continues to generate repetitive bursts of action potentials. Thus the DRG represents the breathing “pacemaker.” Nuclei in this area project primarily to the contralateral spinal cord, where they serve as the principal initiators of phrenic nerve activity (supplying the diaphragm). The DRG also sends many fibers to the ventral respiratory group.
The second group of cells is the ventral respiratory group (VRG). The VRG is located bilaterally in the ventrolateral region of the medulla and is composed of three cell groups (the rostral nucleus retrofacialis, the caudal nucleus retroambiguus, and the nucleus para-ambiguus). The VRG contains both inspiratory and expiratory neurons. The primary function of these neurons is to drive either spinal respiratory neurons innervating the intercostal and abdominal muscles or the upper airway muscles of inspiration. Specifically, inspiratory neurons from the nucleus retroambiguus project to the contralateral external intercostals and to inspiratory and expiratory cells within the medulla, whereas expiratory neurons project to the contralateral spinal cord to drive the internal intercostals and abdominal muscles. The neurons in the nucleus para-ambiguus are primarily vagal motoneurons that innervate the laryngeal and pharyngeal muscles and are active during both inspiration and exhalation. Discharge from the cells in these areas appears to excite some cells and to inhibit other cells. The retrofacialis nucleus consists primarily of expiratory neurons. One group of expiratory cells located most rostrally in the retrofacialis nucleus is called the Bötzinger complex . This is the only group of neurons in the VRG that have been demonstrated to inhibit inspiratory cells in the DRG.
Inspiration and exhalation at the level of the respiratory control center involve three phases—one inspiratory phase and two expiratory (exhalation) phases ( Fig. 10.3 ). After a latent period of several seconds during which there is no activity, inspiration begins with an abrupt increase in discharge in a crescendo pattern from cells in the nucleus tractus solitarius, and the nucleus retroambiguus. This steady ramp-like increase in firing rate occurs throughout inspiration, during which respiratory muscle activity becomes stronger. At the end of inspiration, there is an “off-switch” event that results in a marked decrease in neuron firing, at which point inspiratory muscle tone falls to its preinspiratory level and exhalation begins. At the start of exhalation (phase I), there is a paradoxical increase in inspiratory neuron firing that slows down or “brakes” the expiratory phase by increasing inspiratory muscle tone as well as expiratory neuron firing. This inspiratory neuron firing decreases and becomes absent during phase II of exhalation.

Although many different neurons in the DRG and VRG are involved in ventilation, each cell type appears to have a specific function. For example, within the DRG there are cell populations called Iα cells, which are inhibited by lung inflation, and Iβ cells, which are excited by lung inflation. These two cell populations may be important in the Hering-Breuer reflex , an inspiratory-inhibitory reflex that arises from afferent stretch receptors located in the smooth muscle of the airways. Increasing lung inflation stimulates these stretch receptors and results in an early exhalation by stimulating neurons associated with the off-switch phase of inspiratory muscle control.
Two other areas located in the brainstem are also important in respiratory control. The pneumotaxic center is located in the upper pons in the nucleus parabrachialis medialis and the Kölliker-Fuse nucleus. Impulses from the pneumotaxic center result in premature termination of the inspiratory ramp, resulting in a shortened inspiration. As a result of a shortened inspiration, respiratory rate increases. Thus the pneumotaxic center can regulate tidal volume and respiratory rate. It appears to be important in fine-tuning respiratory rhythm.
The apneustic center is located in the lower pons. When the brain is sectioned just above this area, apneusis , or prolonged inspiratory gasps, are seen if the vagus nerves are also transected. (Apneusis does not occur if the vagus nerves are intact.) Apneusis is probably caused by a sustained discharge of medullary respiratory neurons. Its role in humans is unclear, but it may be the site of afferent information that terminates inspiration. Apneustic breathing is seen after head trauma that damages the pons, and its presence helps localize the site of injury.
Thus rhythmic breathing depends on a continuous (tonic) inspiratory drive from the dorsal respiratory group and on intermittent (phasic) expiratory inputs from the cerebrum, thalamus, cranial nerves, and ascending spinal cord sensory tracts. The DRG may drive the VRG, but contrary to early thought, reciprocal inhibition between the two groups is unlikely.
Spinal Pathways
Axons from the respiratory control center from the cortex and from other supraspinal sites descend in the spinal cord white matter to the diaphragm and to the intercostal and abdominal muscles. These descending axons are coupled with local spinal reflexes in an integrated manner. Descending axons with inspiratory activity excite external intercostal motor neurons and, at the same time, inhibit internal intercostal motor neurons by exciting spinal inhibitory interneurons. Premature infants have uncoordinated respiratory muscle activity, especially during sleep, that can result in paradoxical chest wall and abdominal motion. In addition to contributing to abnormal gas exchange, this paradoxical motion can result in respiratory muscle fatigue and respiratory failure.
Central Chemoreceptors
Chemoreceptors are receptors that respond to a change in the chemical composition of the blood or other fluid around it. Central chemoreceptors are specialized cells that are located on the ventrolateral surface of the medulla but are anatomically separate from the medullary-located respiratory control center ( Fig. 10.4 ). These chemoreceptors are sensitive to the pH of the extracellular fluid around them. Because this extracellular fluid is in contact with the cerebrospinal fluid (CSF), changes in the pH of the CSF affect ventilation by acting on these chemoreceptors.

CSF is in part an ultrafiltrate of plasma that is secreted continuously by the choroid plexus and reabsorbed by the arachnoid villi. Because it is in contact with the extracellular fluid in the brain, CSF reflects the conditions surrounding the cells in the brain. Although the origin of CSF is the plasma, the composition of CSF is not the same as plasma because there is a barrier to free ion flow between the two sites. This barrier is termed the blood–brain barrier , and it separates CSF from the arterial blood. It is composed of endothelial cells, smooth muscle, and pial and arachnoid membranes, and it regulates ion flow. In addition, the choroid plexus also determines the composition of CSF.
The blood–brain barrier is relatively impermeable to hydrogen and HCO 3 – ions, but molecular CO 2 diffuses across it readily ( Fig. 10.5 ). Because of this, alterations in arterial P co 2 are rapidly transmitted to the CSF with a time constant of about 60 seconds. Thus the P co 2 in the CSF parallels the arterial P co 2 . It is not identical, however, because carbon dioxide is also produced by the cells of the brain as a product of metabolism. As a consequence, the P co 2 in the CSF is usually a few torr higher than the P co 2 in the arterial blood, and the pH is slightly more acidic (7.32–7.33) than in plasma.

In addition to being a plasma ultrafiltrate with a slightly lower pH, the P co 2 of CSF is about 10 mm Hg higher than arterial P co 2 and the protein content is considerably lower than plasma (15–45 mg/100 mL, compared with 6.6–8.6 g/100 mL in plasma). As a result of this lower protein concentration, CSF has a lower buffering capacity compared with blood.
Similar to plasma, the Henderson-Hasselbach equation relates the pH of CSF to the bicarbonate ion concentration (HCO 3 – ):
pH=pK+log[HCO3−]0.3×PCO2
An increase in H + ion concentration or P co 2 or both results in a decrease in CSF pH that stimulates the central chemoreceptors, resulting in an increase in ventilation ( Fig. 10.6 ). Ventilation increases almost linearly with changes in H + concentration. Thus the CO 2 in blood regulates ventilation by its effect on the pH of CSF. The resulting hyperventilation reduces P co 2 in the blood and therefore in CSF. Increased arterial P co 2 is also accompanied by cerebral vasodilation that enhances the diffusion of CO 2 into CSF. Although central chemoreceptors are very sensitive to changes in carbon dioxide, central chemoreceptors do not respond to hypoxia.

V ˙
e ). Note that the relationship is linear.
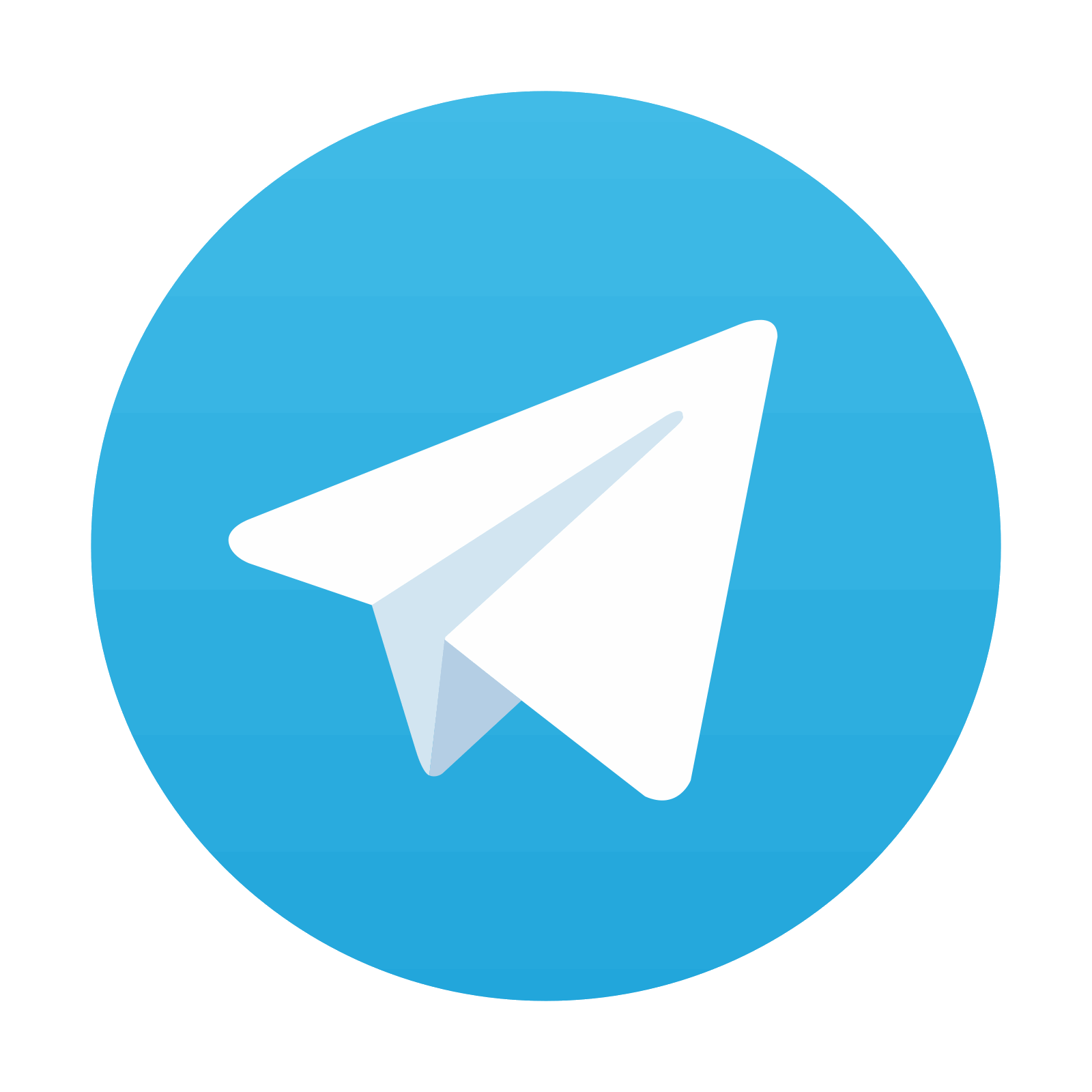
Stay updated, free articles. Join our Telegram channel
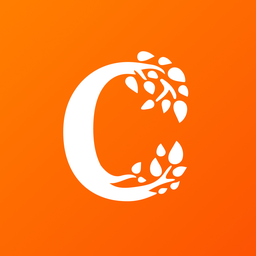
Full access? Get Clinical Tree
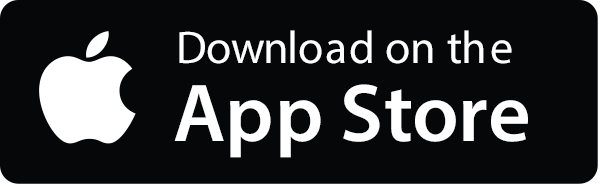
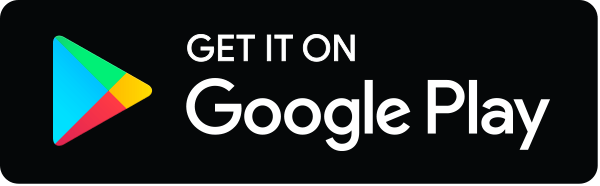
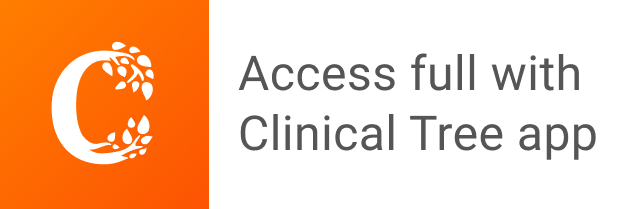