Objectives
- 1.
Describe patterns of particle deposition in the nose and airways and how they relate to lung defense and disease.
- 2.
Describe the role of the nose in lung defense.
- 3.
Describe the three major components of mucociliary transport and their interaction in the defense of the lung.
- 4.
Explain how the lung functions as an organ of the mucosal immune system.
- 5.
Explain the roles of phagocytic, dendritic, and natural killer cells in lung defense.
- 6.
Describe the function of the lung in humoral immune mechanisms.
- 7.
Outline the immune response to bacteria in the normal lung.
- 8.
List four pulmonary diseases associated with abnormalities in mucociliary transport and innate and adaptive immunity.
In addition to its primary function of gas exchange, the lung also functions as a major defense organ to protect the body from the outside world. Just as the respiratory system has developed unique systems to effect gas exchange, it has also developed a unique series of defense systems to cope with environmental exposure and the constant insult of foreign agents.
The respiratory tract is continuously exposed to dust, pollen, ash, and other products of combustion; to microorganisms such as pathogenic viruses and bacteria; to particles or substances such as asbestos and silica; and to hazardous chemicals and toxic gases. In addition, liquids and food particles can be accidentally aspirated (inhaled) from the oropharynx or nasopharynx into the airways. The respiratory tract processes and disposes of these foreign substances using three categories of defense mechanisms: (1) mucociliary clearance that moves inhaled and trapped particles cephalad toward the mouth, (2) phagocytic and inflammatory cells that destroy inhaled material, and (3) a specialized mucosal immune system.
Aerosol Deposition in the Lung
Aerosols are collections of particles that remain airborne for a substantial length of time. Large-particle aerosols tend to settle rapidly to the floor in a sealed room, whereas fine-particle aerosols remain airborne for longer periods of time. In the respiratory tract, the pattern of aerosol deposition is based on particle size ( Fig. 11.1 ), distance traveled, mode and pattern of breathing, size and shape of the airways, particle density, and relative humidity.

There are three major mechanisms of particle deposition in the airways: impaction, sedimentation, and diffusion ( Fig. 11.2 ). Impaction is due to the inertia of particles, causing them be moved toward an airway wall when there is a change in direction of the airstream. (This is similar to the sensation of being pushed against the outer wall of a car when going around a corner at high speed.) In general, particles larger than 5 μm in diameter are deposited by impaction in the nasal passages, where airflow is high and changes direction abruptly as a result of the upper airway anatomy. The anatomy of the nose is ideally suited for this function (see Fig. 1.1 ), with ribbons of tissue ( turbinates ) and nasal hairs ( vibrissae ) over which air is scrubbed. In more distal areas, where airflow is slower, smaller particles (0.2–2.0 μm in diameter) deposit on the surface or on mucus secondary to gravity. This gradual settling of particles based on their weight is called sedimentation . Particle deposition by sedimentation occurs extensively in the small airways, including the terminal and respiratory bronchioles, in large measure because these airways are so small that particles have a short distance to fall, and airflow is so slow. Random movement of particles ( Brownian motion ) as a result of their continuous bombardment by gas molecules is called diffusion and is the third mechanism of particle deposition. Particle deposition by diffusion occurs with particles less than 0.1 μm in diameter and mainly takes place in the smallest airways and alveoli. These particles are cleared by endocytosis by the alveolar macrophages, or they are carried away by lymphatics/lymphoid tissue or transported to the beginning of the mucociliary transport system.

Inhaled Toxicants, Air Pollution, and Disease
Because the respiratory tract is one of the few organ systems in direct contact with the external environment, inhaled toxic materials can cause severe respiratory tract injury. The bronchioles and the alveoli are the most sensitive to inhaled toxicants in part because they are thin (one cell thick) and not coated by a layer of mucus. Protection of these airways from inhaled toxicants represents an important respiratory defense mechanism. A physiologic function of the nose and large airways is to scrub toxicants from the inhaled airstream, a process that limits penetration to the more distal bronchiolar and alveolar airways. The structure and function of the nose and bronchi are well suited for this purpose. This phenomenon occurs for both particulates and gases and vapors.
Particle size is the fundamental basis for the establishment of particulate air pollution standards. The U.S. National PM2.5 (particulate matter 2.5 µm) and PM10 standards are based on potential risks of particles smaller than 2.5 and 10 μm, respectively. In the nasal cavity, impaction is the primary mechanism of particle deposition for particles with diameters greater than 5 μm in diameter. Because of impaction, approximately 50% of inhaled 2.5-μm particles and greater than 90% of 10-μm particles deposit in the nose. Thus approximately one-half of inhaled 2.5-μm particles penetrate through the nose to the lower respiratory tract, whereas less than 5% of 10-μm particles so do. During mouth breathing the nose is bypassed, and there is a large increase in the delivery of inhaled particulates to the lower respiratory tract.
As noted earlier, particles of differing size deposit with differing efficiency within the various regions of the respiratory tract. Less well appreciated is that the nose also scrubs airborne vapors and gases. The same structural and physiologic properties that optimize the ability of the nose to heat and humidify the airstream, specifically narrow airspace, large surface area, and large blood flow, also serve to enhance the scrubbing of vapors and gases. Nasal scrubbing is most efficient for water-soluble, chemically reactive gases and vapors. An extreme example is sulfur dioxide, the ubiquitous air pollutant that is associated with acid rain and derived in large part from combustion of coal. More than 95% of inhaled sulfur dioxide is absorbed in the nose during nose breathing, and less than 5% penetrates to the lower airways. Clearly this serves to protect the lower airways from the harmful effects of such materials.
The protection offered by the nasal cavity is lost during mouth breathing during exercise. Moreover, there is a large increase in ventilation during exercise. These two factors lead to a profound increase in delivery of inhaled materials to the lower respiratory tract during exercise. Consider the pollutant sulfur dioxide. Switching to mouth breathing increases delivery to the lower respiratory tract 20-fold (100% penetration rather than 5% penetration); if accompanied by a 4-fold increase in ventilation, then the total delivered dose to the lower airways would increase 80-fold during exercise. For this reason, persons who exercise outdoors are considered to be at much greater risk from air-pollutant-induced lung injury and diminished lung function than sedentary individuals. This includes not only labor-intensive workers but also children playing outdoor sports and joggers who run in polluted cities.
Analogous to the nose, the bronchial airways also scrub inhaled toxicants from the airstream, a process that limits penetration to the distal bronchiolar and alveolar airways. Few, if any, inhaled particles greater than 10 μm penetrate to the alveoli. In contrast to 10-μm particles, 2.5-μm particles can penetrate to the alveoli; in fact, approximately 25% of inhaled 2.5-μm particles deposit in this region. Thus it can be appreciated that markedly differing alveolar health risks would be anticipated from PM2.5 versus PM10. Gases and vapors can also be absorbed efficiently within the bronchial airways. These airways are considered to be inert (e.g., dead space) with respect to oxygen or carbon dioxide, but this is not the case for soluble and reactive vapors and gases. Such materials are efficiently absorbed in the bronchial airways, resulting in very little penetration to the alveoli. An illustrative example is formaldehyde. This carcinogenic gas is near completely absorbed in the large airways; no inhaled formaldehyde penetrates to the alveoli. As a result, formaldehyde, although it causes large airway injury, does not pose a risk of causing alveolar injury. Diacetyl, the vapor associated with popcorn worker’s lung disease, provides another example of this phenomenon (see Clinical Box: Popcorn Lung Disease).
In the early 2000s an astute physician noted a cluster of individuals with bronchiolitis obliterans among employees in a single microwave popcorn factory. Some workers demonstrated such severe fibrosis of the bronchioles (associated with a forced expiratory volume in 1 second [FEV 1 ] <20% of predicted) that lung transplantation was required. Surveys of the workplace air were performed and revealed the presence of more than 60 hazardous chemicals. A chemical of particular interest, diacetyl (2,3-butanedione), was present in high concentrations. Inhalation toxicity safety evaluation studies in rodents revealed that diacetyl caused nasal and large bronchial, but not bronchiolar, injury. Importantly, this testing was performed in sedentary nose-breathing rodents, a physiologic condition considerably different from exercise with mouth breathing such as that experienced by the workers in the popcorn industry. Subsequent studies revealed that the dose of diacetyl that is delivered to the bronchiolar airways of mouth-breathing exercising workers exceeded that in the sedentary rat by 30-fold or more. Moreover, introduction of diacetyl into the small airways of the rat caused small airway fibrotic disease similar to that observed in workers. It is now accepted that diacetyl causes small airway disease in humans. Diacetyl is the natural chemical that imparts the taste of butter. Diminished lung function has been observed not only in workers in the microwave popcorn industry but also in workers in other occupations that are exposed to butter flavorings, including coffee roasting and certain baking occupations. This exemplifies not only the difficulties in safety evaluation of inhaled materials but also the importance of understanding occupational exposure history in evaluating individuals with lung dysfunction.
Mucociliary Transport
The mucociliary transport system is another one of the lung’s structural, primary defense mechanisms. It protects the conducting airways by trapping and removing bacteria, inhaled particles, and cellular debris from the lung. In general, the longer inhaled material remains in the airways, the greater the probability that lung damage will occur. Once particles in the terminal airways have entered the interstitium, clearance is even slower, and the likelihood of lung damage is even greater.
There are three major components of mucociliary transport: (1) the periciliary fluid, (2) the mucus layer, and (3) the cilia that beat in a lower layer of nonviscid, serous fluid (periciliary fluid) ( Fig. 11.3 ). Effective clearance requires both ciliary activity and respiratory tract fluid (periciliary fluid and mucus). Inhaled material is trapped in the relatively tenacious and viscous mucus, whereas the watery periciliary fluid allows the cilia to move freely with only their tips contacting the mucus and propelling it toward the mouth.

Periciliary Fluid
The periciliary fluid layer is produced by the pseudo-stratified, columnar epithelium that lines the respiratory system and that is joined together by tight junctions (see Fig. 1.10 ). Airway epithelial cells are ciliated and line the entire respiratory tract to the level of the bronchioles, where they are replaced by a cuboidal, nonciliated epithelium. The only exceptions are parts of the pharynx and the anterior third of the nasal cavity. The respiratory epithelial cells are responsible for maintaining the level of the periciliary fluid, a layer of water 5 μm in depth, and electrolytes in which the cilia and mucociliary transport system function. The depth of the periciliary fluid is maintained by the movement of ions across the epithelium. Active (i.e., dependent on energy, as in adenosine triphosphate) chloride secretion into the airway lumen occurs through chloride (Cl – ) channels in the apical membrane. These channels are regulated by intracellular cyclic adenosine monophosphate (cAMP) and calcium. Sodium (Na + ) is absorbed through sodium channels in the apical membrane ( Fig. 11.4 ). Both chloride secretion and sodium absorption translocate water secondarily (osmotic equilibrium), and it is the balance between Cl – secretion and Na + absorption that regulates the depth of the periciliary fluid. A sodium-potassium adenosine triphosphatase (ATPase; Na + ,K + -ATPase) pump in the basolateral membrane maintains the sodium gradient, allowing for sodium absorption. An Na + -Cl – cotransporter in the basolateral membrane links sodium and chloride flux, leading to a buildup in chloride in the cell above its electrochemical equilibrium and to diffusion down a favorable gradient into the airway lumen. The sodium that accompanies chloride is then transported back across the basolateral membrane by the Na + ,K + -ATPase pump.

Thus active secretion of Cl – into the airway lumen produces fluid secretion, whereas active Na + absorption accounts for the ability to absorb fluid. It is the balance between chloride secretion and sodium absorption that regulates the depth of the periciliary fluid at 5 to 6 μm ( Table 11.1 ). This depth is important for the normal functioning of the cilia. If it is too deep, cilia splash around, and if it is not deep enough, ciliary function is markedly diminished.
Agent | Surface ∗ | cAMP |
---|---|---|
β-Adrenergic agonist | Submucosal | ↑ |
Prostaglandin E 2 | Submucosal | ↑ |
Prostaglandin F 2 | Submucosal | — |
Vasoactive intestinal peptide | Submucosal | ↑ |
Adenosine | Mucosal | ↑ |
Leukotrienes LTC 4 and LTD 4 | Mucosal and submucosal | ↑? |
Substance P | Mucosal and submucosal | ? |
Bradykinin | Mucosal and submucosal | ↑? |
∗ Surface is the side of the epithelium on which the agents act.
Mucus Layer
The mucus or gel layer lies on top of the periciliary fluid layer and is propelled by the cilia. Airway mucus is a complex mixture of macromolecules including proteins, glycoproteins, electrolytes, and water. The mucus layer is 5 to 10 μm thick and exists as a discontinuous blanket (i.e., islands of mucus). Three cells produce the mucus layer: surface secretory cells, submucosal tracheobronchial glands, and Clara cells. These cells control both the quantity and composition of macromolecules in the mucus.
Mucus is composed of glycoproteins and consists of groups of oligosaccharides that are attached to a protein backbone like the bones of a fish to the vertebral column ( Fig. 11.5 ). The oligosaccharides are bound to the amino acids by “O” glycosidic bonds (i.e., oxygen links the carbohydrate to the protein), and unlinked proteins form disulfide or peptide bonds. The result is a high-molecular-weight glycoprotein with low viscosity and high elasticity. It is this elasticity that prevents mucus from backsliding during clearance. Mucus is 95% to 97% water.

Surface secretory cells (also called goblet cells ) line the respiratory epithelium and are present in approximately every five to six ciliated cells (see Fig. 1.10 ). They decrease in number between the 5th and 12th lung divisions and disappear completely beyond the 12th tracheobronchial division. They secrete neutral and acidic glycoproteins rich in sialic acid. In response to a chemical signal, goblet cells discharge their stored material by the process of exocytosis, in which membrane-bound storage granules fuse with the plasma membrane and subsequently open to the airway lumen and release their contents. In the presence of cigarette smoke or in individuals with chronic bronchitis, surface secretory cells increase in size and number and extend further down the respiratory tract toward the alveolus. Their output also increases, and there is a change in the chemical composition of their secretions.
Submucosal tracheobronchial glands are present normally wherever there is cartilage; they empty into the airway lumen through a ciliated duct. In patients with chronic bronchitis, these glands are increased in number and size and can extend to the bronchioles. The glands’ secretory component consists of mucous cells near the distal end of the tubule, which is lined by “nonspecified” cells, and of serous cells at the most distal end of the tubule ( Table 11.2 ). Mucous cells contain large, often confluent, electron-lucent granules; serous cells contain small, discrete electron-dense secretory granules. The mucous cells of the submucosal tracheobronchial glands secrete acid glycoproteins, whereas the serous cells secrete neutral glycoproteins and contain lysozyme, lactoferrin, and antileukoprotease. In disease, the chemical composition of the glycoproteins does not change, but there is an increase in the volume of the secretions and a change in the ratio of neutral glycoproteins to acidic glycoproteins that modifies the physical properties of the mucus. This change in viscosity and elasticity affects the subsequent clearance of the mucus. Gland secretion is under parasympathetic, adrenergic, and peptidergic (vasoactive intestinal peptide) neural control. Local inflammatory mediators such as histamine and arachidonic acid metabolites stimulate mucus production.
Serous Cells | Mucous Cells | |
---|---|---|
Granules | Small, electron-dense | Large, electron-lucent |
Glycoproteins | Neutral | Acidic |
Lysozyme, lactoferrin | ||
Hormone | α > β-Adrenergic | β > α-Adrenergic |
Receptors | Muscarinic | Muscarinic |
Degranulation | α-Adrenergic | β-Adrenergic |
Cholinergic | Cholinergic | |
Substance P |
Clara cells are located in the bronchioles and contain granules. Although their exact function is not known, they secrete a nonmucinous material containing carbohydrate and protein. They also appear to play a role in bronchial regeneration after injury.
Healthy individuals produce approximately 100 mL of mucus each day. Although some people even today refer to the “mucous blanket” in the airways, the mucus layer is actually “spotty” and varies in thickness between 2 and 5 μm. Most of the volume of the mucus is absorbed by the ciliated, columnar, epithelial lining cells, with only 10 mL reaching the glottis per day. This mucus is propelled to the back of the throat, where it is swallowed.
Cilia
Cilia are the microscopic hairlike scrubbers of the respiratory system. It is estimated that there are approximately 200 to 250 cilia per cell ( Fig. 11.6 ). They are 2 to 5 μm in length and have a structure that has been preserved through evolution from protozoa. Cilia are composed of nine microtubular doublets that surround two central microtubules and are held together by dynein arms, nexin links, and spokes ( Fig. 11.7 ). This structure is ideally suited for their function. The central microtubule doublet contains an ATPase enzyme that is likely responsible for the contractile beat of the cilium. Coordinated ciliary beating can be detected by the 13th week of gestation.


Cilia beat with a coordinated oscillation in a characteristic biphasic, wavelike rhythm called metachronism ( Fig. 11.8 ). They beat 900 to 1200 strokes/min with a “power forward” stroke and a slow return or recovery stroke. During their power forward stroke, the tips of the cilia extend upward into the viscous layer, dragging it and entrapped particles. On the reverse beat, the cilia release the mucus and are contained completely in the sol layer. Cilia in the nasopharynx beat in the direction that will propel mucus into the pharynx, whereas cilia in the trachea propel mucus upward toward the pharynx, where it is swallowed. Ciliary beating is powered by ATP. The bending of the cilia occurs by the sliding of dynein arms interlinking each microtubule pair. This causes a bending to one side. Cilia beat in a coordinated fashion; this coordination occurs by cell-to-cell ion flow, resulting in electrical and metabolic coordination. The mechanism by which cilia and the adjoining cells communicate is unknown. Ciliary function is inhibited or impaired by cigarette smoke, hypoxia, and infection.

Cough, stimulated by irritant receptors that are activated by inhaled or aspirated foreign material, is also an important protective mechanism. Coughing achieves rapid airflow acceleration and extremely high flow rates and, when coupled with dynamic airway compression, is effective in squeezing and clearing material and mucus from the airways (see Fig. 3.11 ).
When functioning normally, the mucociliary transport system is highly effective. Deposited particles can be removed in a matter of minutes to hours. In the trachea and mainstem bronchi, the rate of particle clearance is 5 to 20 mm/min, whereas it is slower in the bronchioles (0.5–1.0 mm/min).
Phagocytic and Inflammatory Cells
Pulmonary alveolar macrophages and dendritic cells (DCs) are mononuclear phagocytic cells that scavenge particles and bacteria in the airways and in the alveoli. DCs and alveolar macrophages are differentiated cells of the myeloid lineage and are the first nonepithelial cells to contact and respond to foreign substances. They are important components of the innate immune system.
Although B and T lymphocytes are the predominant cells involved in mounting an immune response, DCs are a major cell type for antigen presentation to T cells and are required for a maximum response. The major functions of DCs are to capture, process, and present antigen to T cells as well as to either activate or suppress the T-cell response ( Table 11.3 ).
Capture and process antigen |
Migrate to lymphoid tissues |
Present antigen to lymphocytes via major histocompatibility complex |
Activate lymphocytes and enhance stimulatory response |
Express lymphocyte costimulatory molecules |
Secrete cytokines |
Induce tolerance |
DCs are commonly found from the trachea to the alveoli in the parenchyma of the lung and are usually associated with the epithelium ( Fig. 11.9 ). The upper airways are more densely populated with DCs than the smaller airways in the more peripheral regions of the lung. The anatomic location of these cells correlates well with particle deposition in the airways.

Alveolar macrophages are large, foamy, highly active phagocytic cells derived from myeloid progenitor cells in the bone marrow. Alveolar macrophages have a mean life span of 1 to 5 weeks and are derived from blood monocytes, usually as the result of secondary division by other alveolar macrophages. In inflammatory states such as tuberculosis, blood monocytes can, however, migrate into the lung and differentiate into new alveolar macrophages. Alveolar macrophages are found mostly in the alveolus adjacent to the epithelium and less frequently in the terminal airways and interstitial space ( Fig. 11.10 ). They migrate freely throughout the alveolar spaces and serve as a first line of defense in the lower air spaces. They readily and rapidly (usually within 24 hours) phagocytize foreign particles and substances, as well as cellular debris from dead cells. Once a particle is engulfed, the major mechanisms for killing are typical of phagocytic cells and include oxygen radicals, enzymatic activity, and halogen derivatives within lysosomes. Alveolar macrophages kill foreign material rapidly and without mounting an inflammatory response, which enhances lung defense and contributes to the overall defense system. Rapid phagocytosis inhibits binding of these substances to the alveolar surface and prevents possible invasion into the interstitial spaces and tissue damage.

Polymorphonuclear leukocytes (PMNs) are important cells in lung defense against established bacterial infection of the lower respiratory tract. Although rarely found in normal small airways and alveoli, PMNs are a prominent, histologic feature of a bacterial pneumonia. These cells are recruited to the lung by chemotactic factors released by alveolar macrophages and by products of complement activation. Movement into the lung from the pulmonary vasculature is orchestrated by many factors that mediate the process of adhesion, including integrins on the PMN surface and adhesion molecules on the vascular endothelial cells. PMNs phagocytose and kill invading bacteria by generating products of oxidative metabolism toxic to microbes.
Surface enzymes and factors in serum and airway secretions assist in destroying or detoxifying particles and include lysozymes found primarily in PMNs and known to have bactericidal properties; interferon (IFN), a potent antiviral compound produced by macrophages and lymphocytes; complement , an important cofactor in antigen-antibody reactions; and the bacteriostatic lactoferrin , produced by PMNs and glandular mucosal cells. Antiproteases found in normal lungs are especially important in inactivating the elastase enzymes released by macrophages and PMNs during phagocytosis. The most important of the antiproteases is α1-antitrypsin . Individuals with α1-antitrypsin deficiency lack the ability to synthesize this enzyme and are predisposed to the development of emphysema in their 30s and 40s. Some individuals who smoke produce increased levels of these proteases beyond the capacity of the antiprotease systems, resulting in pulmonary inflammation that leads to degradation of the alveolar septal walls and emphysema.
The transition between the conducting airways and the mucociliary transport system and the terminal respiratory units where alveolar mechanisms are important is the Achilles’ heel in what is otherwise a highly effective defense system, because the risk of particle retention at this location is high. In the occupational lung disease called pneumoconiosis (the “black lung” disease of coal miners) or silicosis (the lung disease caused by inhalation of silica during quarrying, mining, or sandblasting), particle sedimentation occurs in the region of the terminal and respiratory bronchioles. The relatively slow rate of particle clearance in this area provides an opportunity for particles to invoke toxic reactions (in the case of silica) or to leave the airway space and enter the interstitial spaces and invoke less intense fibrotic responses. The terminal respiratory unit is the most common location of airway damage in all types of occupational lung disease. It is also likely that deposition of atmospheric particles such as tobacco smoke in this area causes some of the earliest changes in chronic bronchitis.
Silica dust and asbestos are mineral crystals that cannot be dissolved by the macrophage after phagocytosis. The sharp crystals puncture lysosomal membranes, resulting in intracellular lysosomal enzyme release and cell death. Chemotactic factors released from the dying macrophage cause fibroblast migration and collagen synthesis in the region. Migration of additional macrophages into the region to ingest the dead macrophages occurs, and these macrophages are also killed by the nondissolved, sharp mineral crystals. Their death stimulates additional fibroblast migration and additional collagen synthesis (see the accompanying figure). The end result is that the alveolar macrophage now has localized and concentrated silica or asbestos particles in a region of the lung in association with the development of pulmonary fibrosis, a disease associated with reduced lung compliance, impaired gas exchange, and increased work of breathing.
The fate of the alveolar macrophage is varied. It can be taken up into the mucociliary clearance system, it can die within the alveolus and be phagocytized by other alveolar macrophages, or it can migrate into lymphoid tissue or the lung interstitium.
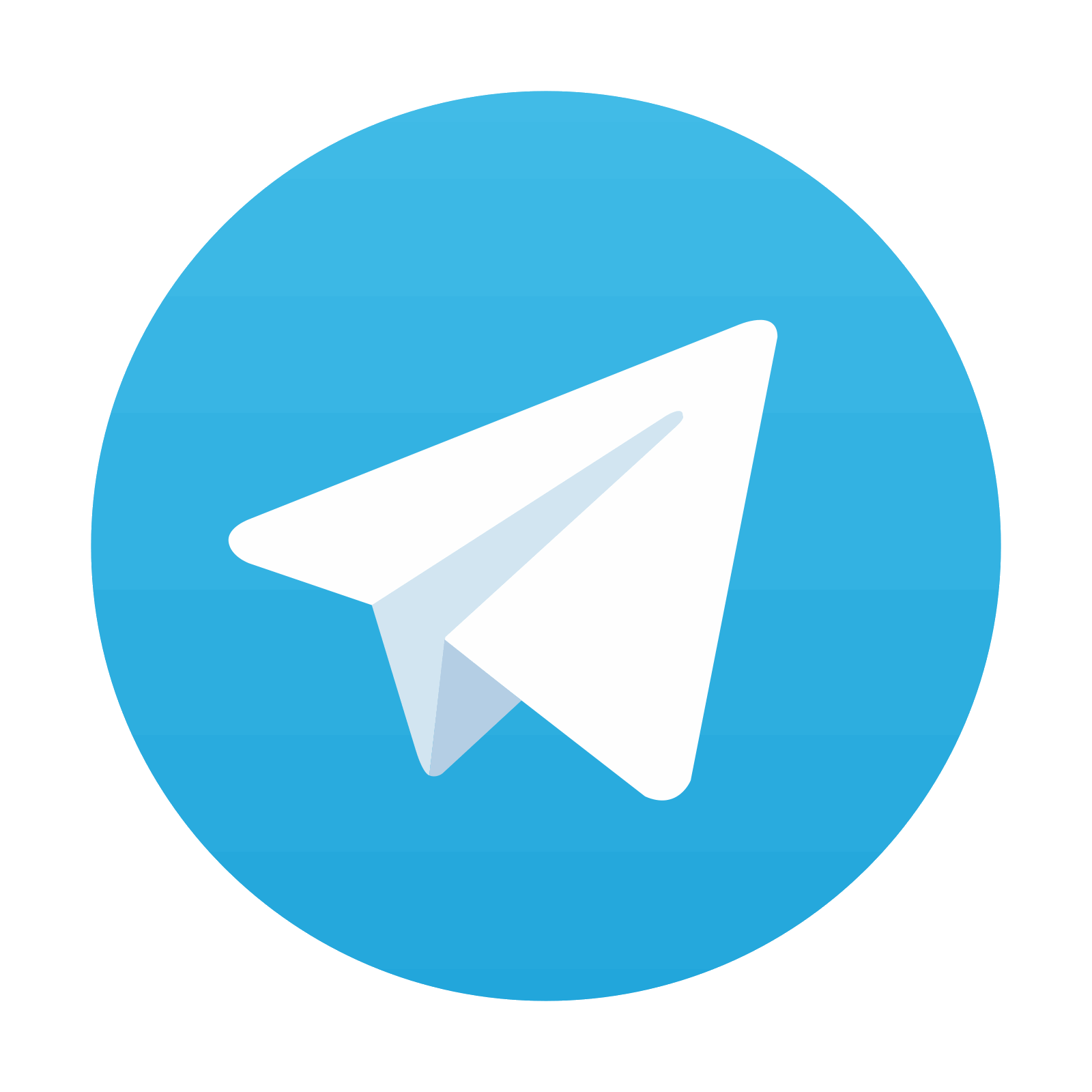
Stay updated, free articles. Join our Telegram channel
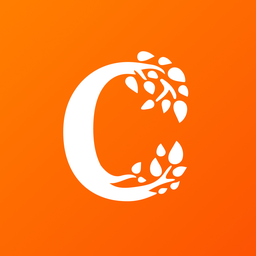
Full access? Get Clinical Tree
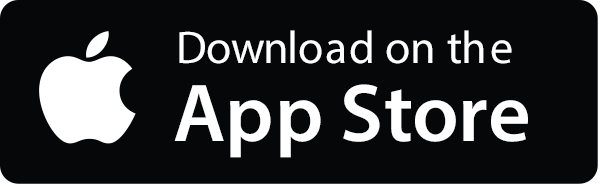
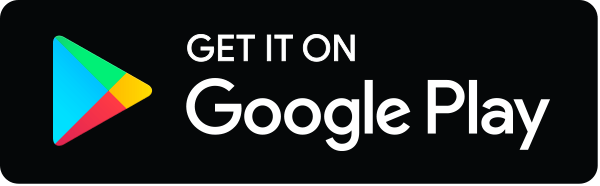
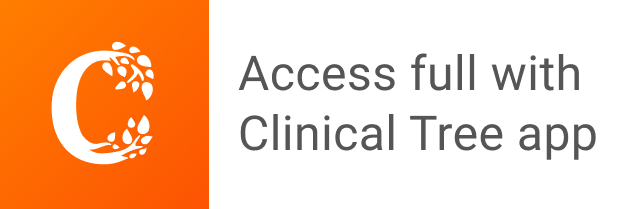