Introduction
Problems with breathing are the root cause of the most common and serious of the sleep disorders. Such breathing problems are associated with a wide range of poor health outcomes including high blood pressure, heart attacks, stroke, diabetes, obesity, and altered brain function. This chapter is structured around several clinically oriented key concepts that relate to understanding the control of breathing and the upper airway during sleep. The key concepts are rooted in basic physiologic principles that are first presented, and these are followed by their application to the spectrum of clinical sleep-related breathing problems. To facilitate understanding of such sleep-related breathing problems further, we present several “perturbations” that importantly impact respiratory function during sleep. We also stress that although each perturbation is defined individually, several such perturbations can coexist in any one person to predispose to respiratory dysfunction. Such respiratory dysfunction can manifest itself either specifically in sleep, or it can be present in wakefulness and made worse by sleep. Ultimately, it is the interaction of these perturbations with mechanisms of respiratory control in sleep that critically impact overall clinical course, stability, and long-term outcome.
Wakefulness and Sleep
Some of the key brain circuits that generate the states of wakefulness, non–rapid eye movement (non-REM) sleep, and REM sleep are summarized in this section. Knowledge of these mechanisms leads to understanding the principle of the “sleep switch.” This principle is then used to explain how the common, serious, and at times life-threatening problems associated with sleep and drug-induced brain sedation and respiratory depression can work through common brain pathways.
Generation of Wakefulness and Sleep
Wakefulness
Figure 85-1A illustrates some of the main neuronal clusters involved in the regulation of brain activity in wakefulness and sleep. Serotonin, noradrenaline, histamine, dopamine, orexin (also named hypocretin), acetylcholine, and glutamate-containing cell groups collectively contribute to the brain activation of wakefulness. Such brain activation manifests as relatively low-voltage and fast-wave activity in the electroencephalogram (EEG), and resting motor tone in the electromyogram recorded from postural muscles.

Some of the neuronal clusters that significantly contribute to the brain activation of wakefulness include dorsal and caudal raphe neurons located in the pons and medulla, respectively (serotonin-containing); the locus coeruleus (noradrenaline-containing); the tuberomammillary nucleus (histamine-containing); the ventral periaqueductal gray (dopamine-containing); the perifornical region of the lateral hypothalamus (orexin/hypocretin-containing); the pedunculopontine and laterodorsal tegmental nuclei in the pons as well as regions of the basal forebrain (acetylcholine-containing); and several of the aforementioned cell clusters plus distributed neurons in what has been commonly termed the reticular formation (glutamate-containing).
Non-REM Sleep
Non-REM sleep is often thought of as the “restorative” nondreaming phase of sleep. It is promoted and sustained by a system of neurons that inhibit the brain-arousal systems of wakefulness (see Fig. 85-1B ). The chief cell groups that comprise this non-REM sleep-active inhibitory system include neurons in the ventrolateral preoptic area and anterior region of the hypothalamus, and regions of the basal forebrain. The cell groups that comprise this non-REM sleep-active inhibitory system synthesize and secrete the inhibitory amino acid gamma-aminobutyric acid (GABA) and the neuropeptide galanin. GABA is one of the chief inhibitory neurotransmitters in the brain. The direct GABA-mediated inhibition of the brain arousal systems identified in the section “ Wakefulness ” and Figure 85-1A , in combination with activation of cortically projecting inhibitory GABA neurons (see Fig. 85-1B ), is responsible for the relatively higher-voltage and slower-wave EEG activity that typifies non-REM sleep.
REM Sleep
REM sleep, on the other hand, is associated with the dreaming phase of sleep and is accompanied by paralysis (atonia) of the skeletal musculature, effects that can also impact breathing. There are two major circuits involved in REM sleep generation ( Fig. 85-2 ). The activation of these circuits produces the defining signs of REM sleep: (1) low-voltage and fast-wave EEG activity and (2) suppression of postural motor tone. Important for the changes in EEG activity is reactivation of the cholinergic cell groups in the pons and basal forebrain that were relatively inactivated during non-REM sleep (see Fig. 85-2 ). The spinal motor activity in REM sleep is suppressed through recruitment of descending neural circuits that involve glycine (principally) and GABA (see Fig. 85-2 ). However, the periods of major suppression of upper airway muscle activity that are also seen in REM sleep do not seem to involve the same mechanism. The genioglossus muscle activity in REM sleep is suppressed through two additional processes: (1) disfacilitation (i.e., withdrawal of excitatory inputs), mediated principally by reduced noradrenaline and serotonin excitation at the hypoglossal motor pool, and (2) inhibition mediated by a newly identified muscarinic receptor mechanism linked to G protein–coupled potassium channels. The latter is illustrated in Figure 85-2 , with the circuitry further explained in “ Breathing and Its Control .”

The “Sleep Switch”
There are mutually opposing interactions between the wakefulness-promoting neuronal systems (see Fig. 85-1A ) and the non-REM sleep-promoting neuronal systems (see Fig. 85-1B ). This organization leads to wakefulness being associated with both a relatively high level of activity in the wake-promoting neuronal arousal systems combined with a relatively low level of activity in the opposing sleep-promoting GABA system (see Fig. 85-1A , panel ii). In contrast, non-REM sleep is associated with both a relatively high level of activity in the sleep-promoting GABA system combined with a relatively low level of activity in the opposing wake-promoting neuronal arousal systems (see Fig. 85-1B , panel ii).
The mutually opposing interactions between the wake-promoting and sleep-promoting neuronal systems produces a situation wherein the brain is in a stable state when either one of the respective neuronal systems dominates. This arrangement constitutes the “ sleep switch ”, in which the position of the switch is stable in either state because when one side is active the alternate state is simultaneously inhibited (see Fig. 85-1 ). In non-REM sleep, for example, the activation of GABA neurons reinforces their own activation via inhibition of the wake-active neuronal systems at the same time.
Effects of Common Neurodepressive Drugs on Brain Arousal State
The reciprocally opposing wakefulness and sleep-promoting neuronal systems identified in sections, “ Generation of Wakefulness and Sleep ” and “ Sleep Switch ,” essentially alter the brain arousal state via the associated changes in the balance of excitatory and inhibitory neurotransmitters in the critical brain regions identified in Figure 85-1 . The balance of these brain neurochemicals, however, is not only changed across natural states of wakefulness and sleep, but also the balance is changed in predictable ways by the ingestion of commonly used neurodepressive drugs. Such drugs include benzodiazepines, imidazopyridines (nonbenzodiazepine sedative hypnotics), barbiturates, ethanol, and some general anesthetics that are either inhalational (e.g., isoflurane) or injectable (e.g., propofol or etomidate). All these agents interact with binding sites on GABA A receptors that enhance neuronal inhibition at sites where GABA would otherwise be acting. There are several sites within the endogenous sleep-wake circuitry identified in Figure 85-1 where such neurodepressive drugs act to depress the brain arousal state and promote sedation and/or loss of consciousness. Such agents, therefore, effectively “tip the balance” within the endogenous sleep-wake circuitry “toward” sedation and “away” from brain arousal.
The principle of the sleep switch can also be used to understand that the depression of brain arousability that is seen in both natural sleep and with neurodepressive drugs is the product of two mechanisms. The first is the augmentation of inhibitory GABAergic influences and the second is the concomitant depression of arousal-related stimulatory influences (see Fig. 85-1B , panel ii). There is a dual effect because these inhibitory and excitatory mechanisms cannot operate independently because of their inherent interconnectedness. This principle has further implications for understanding sleep- and drug-induced respiratory depression.
Application to Sleep and Drug-Induced Respiratory Depression
The organization of the sleep switch and its application to understanding sleep and drug-induced brain sedation (see “ Effects of Common Neurodepressive Drugs on Brain Arousal State ”) also has direct applications to understanding respiratory depression. The respiratory network and its associated control systems are themselves influenced by the same state-dependent arousal/sleep systems illustrated in Figures 85-1 and 85-2 . Such wakefulness and sleep-dependent neuronal systems also have significant projections to the respiratory network.
Accordingly, during both natural sleep and in the presence of many commonly used sedative and anesthetic drugs, there is both augmentation of the GABAergic inhibitory system and corresponding reductions in excitatory influences from the brain arousal systems (as outlined in “ Effects of Common Neurodepressive Drugs on Brain Arousal State ”). The net result is a tipping of the balance in the sleep switch toward low brain arousability plus alteration of drives to the respiratory network. As further outlined in “ Breathing and Its Control ,” these state-dependent drives affect the musculature particularly of the upper airways, thus predisposing to obstructive sleep apneas and hypopneas in susceptible individuals. In essence, the brain functions as a gain-setting device for breathing by altering brain neurochemistry of the key elements of respiratory control: the respiratory neurons, the motoneurons, and the sites involved in the reflex modulation of breathing (see “ Breathing and Its Control ”).
Breathing and Its Control
The complexity of the brain-stem respiratory network can be distilled to three essential elements:
- 1.
Respiratory neurons that generate respiratory rhythm and drive the expression of rhythmic activity in other components of the respiratory network.
- 2.
Respiratory motor pools that innervate and activate the primary and secondary muscles of breathing. The primary respiratory muscles are so designated because they generate airflow (e.g., the diaphragm). In contrast, the secondary (accessory) muscles of breathing do not generate airflow but either significantly modulate its passage (e.g., pharyngeal muscles that maintain a patent upper airway) or otherwise support the act of breathing (e.g., the intercostal muscles that contribute to the maintenance of lung volume).
- 3.
Chemosensors that detect alterations in blood gases and elicit a physiologic response. In the case of hypoventilation during sleep, for example, the appropriate physiologic response includes both an attempted chemoreceptor-mediated increase in ventilation and arousal from sleep. The importance of arousal from sleep is critical to survival in some situations when the ventilatory response is futile by itself, for example, shaking off a suffocating blanket in the case of a sleeping infant to prevent asphyxia and risk of sudden infant death syndrome.
The following sections identify the key circuits that generate breathing and control its regulation.
Respiratory Neurons
Organization
Figure 85-3 illustrates the main components of the respiratory network generating respiratory rhythm and motor activation. The ventral respiratory group (VRG) contains Bötzinger complex (expiratory) neurons, pre-Bötzinger complex (inspiratory) neurons, rostral ventral respiratory group (predominantly inspiratory) neurons, and caudal ventral respiratory group (predominantly expiratory) neurons. The dorsal respiratory group (DRG) contains mainly inspiratory neurons. The DRG and its associated regions of the nucleus of the solitary tract are also the projection sites for afferents important to the reflex control of breathing: the carotid and aortic chemoreceptors and baroreceptors, and lung vagal afferents.

The brain stem also includes motoneurons of the hypoglossal and trigeminal motor nuclei that innervate muscles important to the maintenance of upper airway patency (see Fig. 85-3 ). Regions of the nucleus ambiguus also contain motoneurons that innervate the muscles of the larynx and pharynx via the vagus, glossopharyngeal, and accessory nerves. Respiratory neurons in the pons (not shown) also modulate the activity of medullary respiratory neurons.
Respiratory Rhythm and Motor Activation
Figure 85-3 also illustrates that some respiratory neurons are identified as propriobulbar, that is, neurons that project to, and influence the activity of, other medullary respiratory neurons but themselves do not project to motoneurons. Others are identified as bulbospinal respiratory pre-motoneurons, that is, neurons that project to spinal motoneurons, which in turn innervate the respective respiratory pump and abdominal muscles of breathing.
Inspiration
A particularly important propriobulbar respiratory neuronal group is the pre-Bötzinger complex. This region plays a key role in generating the fundamental respiratory rhythm in mammals. Pre-Bötzinger complex neurons drive activation of bulbospinal neurons of the DRG and VRG during inspiration, which then activate spinal inspiratory phrenic and intercostal motoneurons (see Fig. 85-3 ). Inactivation of pre-Bötzinger complex neurons in animal models leads to ataxic breathing and central apneas, especially in sleep. The pre-Bötzinger complex is also a critical site mediating respiratory rate depression by opioids.
Expiration
In expiration, the expiratory neurons of the Bötzinger complex inhibit inspiratory pre-motoneurons and motoneurons (see Fig. 85-3 ). Also, in expiration, the caudal ventral respiratory group neurons increase excitability of spinal expiratory motoneurons (see Fig. 85-3 ), although this excitation does not necessarily manifest as demonstrable expiratory muscle activation at rest.
Respiratory Rhythm Generation and Central Apneas
The automatic and nonconscious rhythm of breathing is ultimately generated by the intrinsic membrane properties and connections of the individual neurons that comprise the respiratory network. Essential to expression of this rhythmicity, however, is provision of a sufficient level of underlying tonic excitation. Key sources of tonic excitation are from the aforementioned wakefulness-dependent neural systems (see Fig. 85-1 ) as well as the peripheral and central chemoreceptors ( Fig. 85-4 ).

An important physiologic principle that emerges from identification of the necessity and sufficiency of tonic excitation for respiratory rhythm generation is that removal of such tonic excitation can abolish respiratory rhythm. Sleep or neurodepressive drugs (see Fig. 85-1 ), or reduced chemoreceptor inputs (see “ Breathing Is Dependent on Feedback Regulation in Sleep ”), can each lead to cessation of respiratory rhythm and development of central sleep apneas.
Respiratory Motor Pools and Muscle Activity
Respiratory Muscles Vary in the Degree of Their Relationship to Breathing
Different respiratory muscles express varying degrees of respiratory-related activity and/or tonic (i.e., nonrhythmic, continuous, or background) activation. The diaphragm, for example, is almost exclusively respiratory-related while the palatal, tongue, intercostal, and abdominal muscles express both respiratory and tonic activities, to varying degrees. The physiologic basis for this variation is that the respiratory neurons that ultimately drive the respective respiratory muscles themselves vary in the strength of their relationship to breathing. Some respiratory neurons, for example, are almost exclusively driven by respiratory rhythm-generating neurons and are weakly influenced by other inputs. In contrast, there are some respiratory neurons that are weakly influenced by respiratory rhythm-generating neurons but more strongly influenced by tonic nonrespiratory inputs. Such tonic nonrespiratory inputs can arise from the sleep state–dependent cell groups identified in Figure 85-1 .
Effects of Sleep
The identification of respiratory neurons and motoneurons that vary in the strength of their relationship to breathing assumes greater significance because of the physiologic principle identified by John Orem from Texas Tech University. Those respiratory neurons that are most strongly driven by respiratory rhythm-generating neurons are least affected by the transition from wakefulness to non-REM sleep. In contrast, those respiratory neurons that are more strongly influenced by tonic nonrespiratory inputs are most affected by the change from wakefulness to sleep. In the latter case, activity within certain respiratory muscles can cease or be markedly depressed during sleep, especially those showing prominent tonic muscle activity in wakefulness, such as the muscles of the upper airway and chest wall (see Fig. 85-3 ). The key physiologic and clinical implications of this effect are identified in “ Upper Airway Motor Tone and Compensatory Reflex Responses are Particularly Sensitive to Depression In Sleep ”; the loss of the tonic input to muscles of the upper airway is one of the major reasons for the suppression of upper airway muscle activity in sleep and the susceptibility to obstructive sleep apneas.
Chemosensors and Chemoreflexes
Location and State-Dependence of Responses
Arterial O 2 and CO 2 levels are regulated by peripheral chemoreceptors located at the bifurcation of the common carotid arteries, and central chemoreceptors located in the brain. More specifically, the central chemoreceptors are located close to the ventral surface of the medulla in the caudal region of the retrotrapezoid nucleus (see Fig. 85-4 ). Cells in this region of the retrotrapezoid nucleus are intrinsically sensitive to changes in CO 2 /H + . These CO 2 /H + sensitive neurons have dendrites that extend to the ventral medullary surface by which they sense the pH of the surrounding cerebrospinal fluid. They also have axons that project to the rostral region of the VRG by which they drive respiratory network activity.
In addition to the retrotrapezoid nucleus, some of the wakefulness-active/sleep-inactive cell groups identified in Figure 85-1 , such as serotonergic and noradrenergic neurons, are also responsive to alterations in CO 2 /H + levels (see Fig. 85-4 ). For any given inspired CO 2 , the activity and responsivity (i.e., slope of response) of such neurons is reduced in sleep compared to wakefulness. As identified in later sections, this change in cell activity in response to hypercapnia from wakefulness to sleep parallels the change in overall ventilatory response.
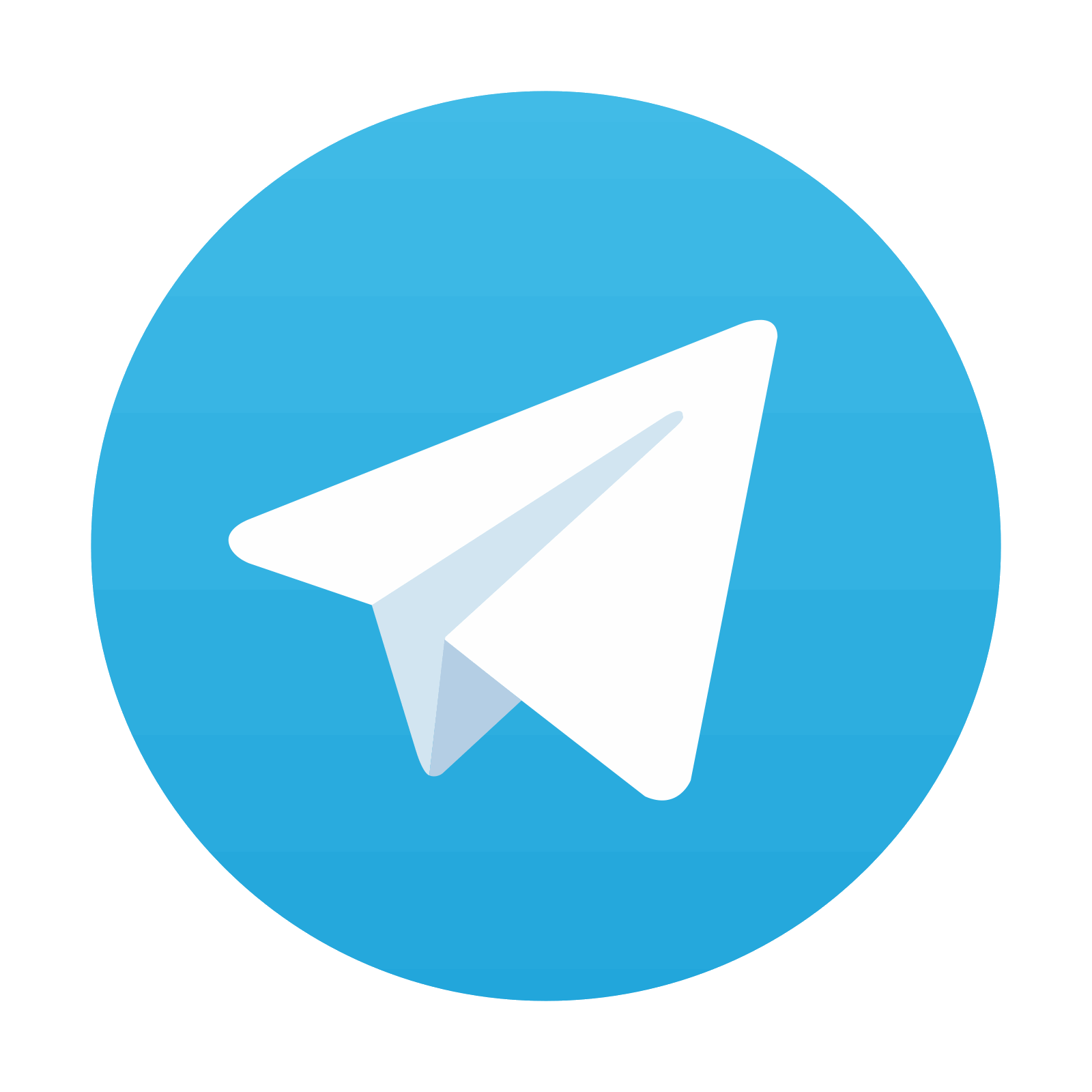
Stay updated, free articles. Join our Telegram channel
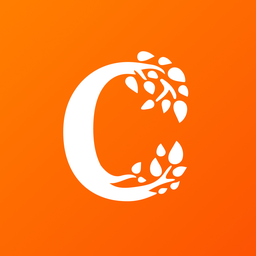
Full access? Get Clinical Tree
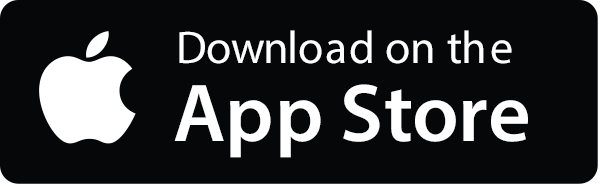
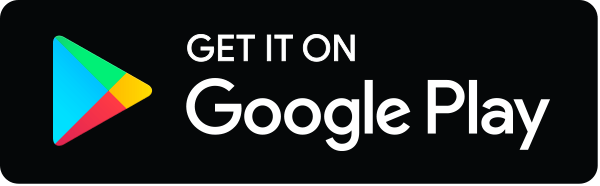