Computed Tomography of the Heart
Ross T. Murphy
Mario J. Garcia
Historical Perspective
The ability to rapidly obtain cross-sectional images of the body with x-ray computed tomography (CT) has revolutionized medicine since its introduction as a clinical tool in 1972. Although the physical principles behind CT were described as early as 1917 by Radon, credit for the technical development is owed to Hounsfield and Cormack, who received the Nobel Prize in Physiology or Medicine in 1979. More recent advances have led to the development of electron-beam CT (EBCT) and multidetector CT (MDCT), which make it possible to obtain electrocardiogram (ECG)-gated images with sufficient temporal and spatial resolution to visualize the beating heart. The first clinical applications of cardiac EBCT in the 1980s were focused on the evaluation of cardiac volumes and function, the pericardium, and the great vessels. Subsequently, this technology gained popularity for the detection and quantification of coronary calcifications. The first cardiac application of MDCT followed the introduction of four-detector scanners in the late 1990s. In addition to evaluating coronary calcifications, both EBCT and MDCT have demonstrated the capability of performing contrast-enhanced coronary angiography (1,2,3,4,5,6,7). The evolution of MDCT technology has occurred much faster than that of EBCT over the last decade. State-of-the-art MDCT technology represents the seventh generation of CT scanners and has overcome many of its previous limitations. Image quality is undergoing constant refinement, and the number of uninterpretable coronary studies has gradually decreased from 20% to 40% using 4-detector systems, to 15% to 25% with 16-detector systems, to as low as 3% to 10% with 64-detector systems.
Physical Principles of Computed Tomography
Image Acquisition
The clinical value of cardiac CT was quite limited prior to current iterations of CT technology. Cardiac imaging demands a very high temporal resolution because the heart is in constant, rapid phasic motion with translational movement and torsion, which have been difficult to capture even with high-frame-rate echocardiography until recently. For the purpose of coronary imaging, submillimeter spatial resolution is also needed because of the diameter of the coronary vessels.
The physics of EBCT and MDCT differ significantly, but they share some common principles: (a) an x-ray source is required to produce the images, (b) a detector crystal receives the x-rays after they travel through the imaging target and converts photons into electrical impulses, (c) x-ray data need to be collected for a minimum of 180° to form a cross-sectional image, (d) the in-plane pixel dimensions define the x- and y-axis resolution, but the slice thickness determines the z-axis resolution, (e) multiple cross-sectional images need to be acquired to form a three-dimensional volume, and (f) advanced computing power is used to reconstruct a three-dimensional data set with a unique set of x, y, and z locations corresponding to each target of information collected, using a standard technique called filtered backprojection. In addition, cardiac CT requires temporal registration to match data collected at the same time of the cardiac cycle because of the continuous motion and deformation of the heart.
With EBCT, a rapidly rotating electron beam generates x-rays without the need for mechanical rotating parts. This technology was developed specifically with cardiac imaging in mind. A stationary high-voltage electron gun produces a beam of electrons, which is rapidly steered to sweep over tungsten targets arranged in a semicircular array under the patient table. Thus a fan of x-rays is created that penetrates the patient. Stationary detector arrays receive the attenuated signal. Cross-sectional images thus can be rapidly acquired without the constraints of mechanical motion: The electron beam can sweep across the target rings very rapidly in one rotation providing temporal resolution as fast as 33 to 50 msec. A limitation of EBCT technology is its relatively slow speed of data collection in the z-axis because only one slice of data can be collected at a time. This results in limited spatial resolution in the z-axis and/or prolonged scan acquisition time. In addition, x-ray power delivery is fixed and limited, resulting in noisier images, in particular when imaging obese patients.
![]() FIGURE 56.1. Diagram of the rotating gantry of a multidetector computed tomographic system. A: X-ray tube. B: Precollimator. C: Target (body). D: Postcollimator. E: Multidetector array. |
MDCT imaging involves an x-ray source that rotates around the patient and emits a fan-shaped beam of x-rays that passes through the body at many angles (Fig. 56.1). Collimators are used to confine the x-ray beam to the slice to be imaged. Current-generation MDCT scanners use a spiral or helical scanning mode that offers faster coverage of larger volumes than older “stop and shoot” scanning, but they require that the x-ray tube and the detector array be rapidly rotated around the body. While the gantry (with x-ray tube and detectors) is rotating and data are being acquired continuously, the table where the patient lies supine is advanced at a constant speed. After the x-ray data set has been collected, images can be reconstructed retrospectively. To reconstruct an image at a given level, a set of projections from numerous angles needs to be acquired at that level. Because the tube does not perform a full rotation in any given plane, x-ray data for every projection angle are “interpolated” with data from the rotation before and after. Recent advances include a “slip ring” so gantry rotation is wireless, mathematical filtering of the acquired data with a filter or “kernel,” and complex algorithms for digital image display. Multiple-detector arrays have been introduced to clinical practice. Commercially available state-of-the-art MDCT scanners have up to 64-detector arrays, but 128- and 256-detector scanners are already in use in a research setting. The simultaneous acquisition of multiple cross-sectional images makes it possible to do a complete acquisition of the heart in a single 8- to 12-second breath-hold. One limitation of MDCT is its lower temporal resolution (175 to 220 msec), which is determined by the rotational speed of the gantry. This can result in motion artifacts when imaging the coronary arteries. Thus, most studies require the administration of β-blockers to reduce the patient’s heart rate during acquisition. However, a novel algorithm can be implemented to collect data from different detector arrays to form a single slice. These multicycle reconstruction algorithms can often improve temporal resolution to 80 to 120 msec but require a very steady heart rate and rhythm.
Image Reconstruction
Although cardiac CT provides continuous cross-sectional images of the heart, every displayed image must be synchronous with and constructed from the same phase of the cardiac cycle. If this is not done, gaps in the data set and/or temporal misregistration artifacts may render the study useless. Data acquisition can be prospectively triggered by the patient’s ECG. In this mode, the data are acquired intermittently at a time determined by the position of the QRS. Prospective ECG gating is mostly used during EBCT scanning. Alternatively, data can be acquired continuously together with the ECG signal, and then portions of the data set corresponding to specific phases of the cardiac cycle can be reconstructed to form a static three-dimensional image. This method, referred as retrospective ECG gating, involves greater radiation exposure because the x-ray tube is being used continuously, but it provides more-consistent image quality if irregularities in the heart rhythm occur. As a general rule, however, imaging of the coronary arteries is unreliable in patients with irregular rhythms. To reconstruct a complete cross-sectional image, data collected from projections over 180° plus the width of the fan angle emitted by the x-ray tube (approximately 50°) are required. Image reconstruction algorithms that use projections covering less than 360° are called partial scan reconstruction algorithms. With this reconstruction technique, a temporal resolution of about one half of the gantry rotation time can be achieved in the center of the scan field. To fill gaps and provide missing projection angles for reconstruction of an image at a given level, data are used that are acquired during a later heart beat by another detector and assigned to the correct heart phase by means of the simultaneously recorded ECG.
Image Display
Each pixel of the image to be reconstructed is assigned an x-ray attenuation value expressed in Hounsfield units (HU). The Hounsfield scale is a prespecified score with values of 0 HU for water, –1,000 HU for air, and approximately +1,000 HU for bony cortex, with a range from –1,024 to 3,071 HU. Because the human eye cannot distinguish gray scale over such a wide range, adjustments (window “width” and window “level” or “center”) are made to analyze the reconstructed images. The “width” value is the range of gray-scale CT numbers to be displayed. Each value below that range is shown black; each value above the range is shown white. The “level” or “center” value determines the CT number on either side of which the “window” is fixed (thus in an image display with a center of 600 HU and width of 400 HU—i.e., ±200, each pixel with a density of <400 HU will displayed black and each pixel with a density of >800 HU will be displayed white). Soft tissue, including muscle, and nonenhanced blood have a score
ranging from –100 to +200, but arterial blood with contrast has a score of +200 to +400.
ranging from –100 to +200, but arterial blood with contrast has a score of +200 to +400.
Once transformed and reconstructed, the CT data set can be displayed in a number of different ways. These postprocessing protocols include the following:
Axial image assessment. This should be the first step in any assessment of acquired study. Scrolling up and down though the originally acquired cross-sectional images is useful to assess for normal anatomy, chamber, and vessel relationships.
Multiplanar reconstruction (MPR) images are reconstructed from the three-dimensional data set in any coronal, sagittal, or oblique orientation. This is relatively simple with the new-generation MDCT scanners because they provide isotropic resolution.
Curved multiplanar images are on a plane reformatted to fit a curve (usually following a coronary artery) and allow the display of the entire structure in a single image. Although this creates some distortion, it is a useful way to analyze a tortuous vessel. Careful adjustment of image center and window levels is useful to differentiate the contrast-enhanced lumen from calcified and noncalcified coronary plaques. This procedure is repeated for each vessel and its branches.
Maximum intensity projection (MIP) images smooth out the projection of three-dimensional images and assign the maximum detected value to that portion of the image, thus projecting the highest attenuation values in that two-dimensional direction, a little like conventional coronary angiography. Obscuring lower-density structures highlights contrast-filled vessels over other structures. The “slab” image may thickened at will to smooth out planes, and is also useful for displaying long segments of vessels.
Volume-rendered images. These images use all the volumetric data acquired in the scan and combine the “voxels” (volume elements) into a three-dimensional image. These images are useful for assessing the relationships between different structures. Coloring the image often provides a “realistic” anatomic view.
Reconstructions combining set fixed points in the cardiac cycle (0% of the RR interval + 10% + 20%…) can generate a cine film. Atrial and ventricular volumes and ejection fraction can be then quantified from the four-dimensional data set.
Radiation Dose and Patient Safety
Cardiac CT, like invasive angiography, involves radiation exposure. The “effective dose,” expressed in millisieverts (mSv), depends on multiple factors including volume of acquisition required, duration of the scan, and radiation energy level. The volume of acquisition is typically 12 to 16 cm for coronary angiography and 18 to 25 cm for angiography for coronary bypass conduits. The radiation energy level required to obtain adequate image quality also depends on the weight of the patient. Obese individuals require larger amount of energy due to scattering and attenuation. Current-generation systems with 64 detectors provide a typical dose range in the order of 4 to 12 mSv for MDCT coronary angiography. This compares to 2 to 6 mSv for invasive angiography, 10 to 20 mSv for nuclear stress tests involving thallium, and 3.6 mSv from yearly background radiation exposure. EBCT examinations result in an effective dose of about 1.1 to 2.0 mSv. Strategies to minimize the effective dose in MDCT include dose modulation, in which the x-ray dose is stepped up in the ECG-gated target area in mid-diastole and stepped down in the rest of the cardiac cycle. This can reduce total radiation exposure by up to 50% and should be used whenever possible in coronary MDCT. The estimated risk of cancer increases by 1:2000 MDCT coronary studies. Studies suggest that the longitudinal risk of cancer secondary to radiation exposure decreases with age and is significantly higher in pediatric patients and lower in older adults. Because of these concerns, the indiscriminate use of MDCT as a screening test is not warranted at present, and professional society guidelines for the appropriate use of this technology are awaited.
Clinical Applications
Calcium Scoring
Electron-beam computed tomography scanners have been in clinical use for more than a decade and are used to detect and quantify coronary artery calcifications. Designed with cardiac motion in mind, they can acquire a full data set in as little as 50 msec. The most widely used measure of calcium burden is the calcium score, based on a radiographic density-weighted volume of plaques with pixel numbers of a least 130 HU. The prognostic value of calcification scores has been established (8). Coronary calcification is a robust predictor (the relative risk [RR] for a calcium score of >100 is 1.88) of hard cardiovascular outcomes (death and nonfatal myocardial infarction) at 7 years follow-up. Although very high calcium scores impart an approximate 10-fold increased risk, they do not always imply a tight coronary stenosis. The role of EBCT screening of asymptomatic individuals is controversial, and a study of the incorporation of this type of investigation into a comprehensive risk screening with CRP and cholesterol measurements is ongoing. There is some evidence to support the incorporation of calcium scores into an overall risk stratification of older individuals using clinical algorithms such as the Framingham Risk Score. In the South Bay Heart Watch study (9), a calcium score of greater than 300 was associated with a significant increase in coronary heart disease (CHD) event risk compared with that determined by clinical score alone. These data support the hypothesis that high coronary artery calcium score (CACS) can modify predicted risk, especially among patients in the intermediate-risk category in whom clinical decision-making is most difficult. Those at low risk by clinical score derived no additional benefit from calcium scoring. However, the use of EBCT to improve cardiovascular risk prediction in a population with no cardiac symptoms who are at low absolute risk is very expensive. Some authorities (10) even suggest that EBCT may in aggregate have a detrimental effect on the quality of life of screening populations.
Computed Tomography Coronary Angiography
Without doubt, the ability of providing anatomic information about the coronary arteries is one of the most exciting and potentially important applications in cardiac CT. Adequate training for conducting and interpreting CT coronary studies is absolutely essential to obtain adequate results. Guidelines for training were recently issued by the American College of Cardiology and American Heart Association (11). Neither experience in computed tomography nor that in invasive coronary angiography alone is sufficient to provide the required training. Accordingly, the best results are obtained by the joint effort of cardiologists and radiologists providing complementary expertise.
Contrast-enhanced EBCT can define the coronary anatomy and quantification of stenosis but is limited by poor visualization of distal vessels, and excess calcification can obscure lumen definition. A meta-analysis (12) of the 10 published studies comparing EBA to coronary angiography shows a sensitivity
and specificity of 87% and 91%, respectively, for the detection of obstructive coronary atherosclerosis. An important number (8% to 25%) of the coronary segments, however, were deemed uninterpretable in these studies.
and specificity of 87% and 91%, respectively, for the detection of obstructive coronary atherosclerosis. An important number (8% to 25%) of the coronary segments, however, were deemed uninterpretable in these studies.
Appropriate patient selection is important for obtaining adequate results (13). A careful review of the medical history is important before performing each procedure, including knowledge about prior allergy to iodine contrast, renal function, heart rate, and regularity of rhythm. Adequate oral hydration is encouraged before and after performing the study. A stable, low heart rate is most important during scanning because this determines the target for ECG-gated image reconstruction and sometimes the target for dose modulation (see later discussion). We use intravenous β-blockers administered just before the study and aim for a resting regular heart rate of 50 to 55 beats per minute. In most patients with a heat rate below 60 beats per minute, the time of least coronary artery motion is centered on 75% of the RR interval, corresponding to the diastasis phase of diastole. At higher rates, from 80 to 90 beats per minute, diastasis disappears; therefore image reconstruction at about 40% of the cardiac cycle is preferred. These phases are not merely coincidental with cardiac stasis but also represent the optimal multiple of the gantry rotation plus fan angle for each manufacturer, using reconstruction algorithms. Thus in scanners capable of performing multicycle reconstruction, which provide effective temporal resolution of 100 to 120 msec, adequate coronary visualization may be obtained in many patients with stable heart rates up to 80 to 95 beats per minute (14). Nevertheless, there is significant patient-to-patient variability, and examination of several phases reconstructed at 5% to 10% intervals from 40% to 90% of the RR is sometimes needed. Some centers administer oral β-blockers the night before the procedure. β-Blockers also reduce heart rate variability during the scan, and for that reason we recommend their administration almost routinely unless contraindicated.
Once adequate heart rate is achieved and sublingual nitrates are given, data acquisition is started. In many centers, a calcium-score scan (with no contrast and low radiation of 1 to 2 mSv) is performed first. This is followed by a scout scan (planar x-ray mode), which will be used to select the region of interest (usually from the carina to slightly below the diaphragm, but from the subclavian artery down if an internal thoracic graft is be assessed). The survey scan should be acquired during a brief inspiratory breath-hold.
An iodinated contrast agent is injected to increase the CT attenuation of the blood pool (CT imaging of the heart can be performed without injection of a contrast agent, usually to assess calcified structures within the heart, most notably coronary artery calcification). A remotely controlled dual-injection system capable of administering iodine contrast and saline separately is used. Correct timing of the actual CT scan relative to contrast injection is important. CT scanning can start when the contrast agent reaches a prespecified threshold density in the descending aorta. This is usually 15 to 25 seconds after the start of contrast injection into a peripheral vein. If only arterial structures (left ventricle, coronary arteries) are to be investigated, acquisition can occur 6 seconds after threshold. If structures of the right heart and pulmonary circulation are also to be analyzed, acquisition is adjusted to start later.
Figures 56.2 and 56.3 demonstrate examples of normal and severely stenosed coronary artery. It is important to understand from the outset that MDCT gives a qualitatively different view of the coronary arteries than invasive angiography because the images show Glagovian remodeling and extraluminal atherosclerosis. It is thus facile to expect exact equivalence
between the two imaging modalities. A number of important studies (15,16,17,18,19,20,21,22,23,24) investigated the accuracy of 16- and 64-slice MDCT coronary angiography for the detection of coronary artery stenosis in patients with known or suspected coronary artery disease (CAD) referred for invasive coronary angiography (Table 56.1). In all these studies, analysis of the MDCT data was performed by investigators blinded to the results of invasive angiography, and in all but two, the study was limited to coronary segments of greater than 1.5 or 2 mm in diameter. In most cases, significant coronary artery stenosis was defined as a lesion of 50% or greater, and sensitivity, specificity, and positive and negative predictive values were calculated. The prevalence of significant CAD in patients enrolled in these studies ranged from 42% to 83%. It is important to note that about 5% to 20% of all analyzable segments were considered nonevaluable due to motion, severe calcified plaques, and other imaging artifacts. In addition, most of these studies did not enroll a clinical series of truly consecutive patients, and only a few reported the accuracy of MDCT using each patient as the unit of analysis. Moreover, these studies were performed in few selected single centers.
between the two imaging modalities. A number of important studies (15,16,17,18,19,20,21,22,23,24) investigated the accuracy of 16- and 64-slice MDCT coronary angiography for the detection of coronary artery stenosis in patients with known or suspected coronary artery disease (CAD) referred for invasive coronary angiography (Table 56.1). In all these studies, analysis of the MDCT data was performed by investigators blinded to the results of invasive angiography, and in all but two, the study was limited to coronary segments of greater than 1.5 or 2 mm in diameter. In most cases, significant coronary artery stenosis was defined as a lesion of 50% or greater, and sensitivity, specificity, and positive and negative predictive values were calculated. The prevalence of significant CAD in patients enrolled in these studies ranged from 42% to 83%. It is important to note that about 5% to 20% of all analyzable segments were considered nonevaluable due to motion, severe calcified plaques, and other imaging artifacts. In addition, most of these studies did not enroll a clinical series of truly consecutive patients, and only a few reported the accuracy of MDCT using each patient as the unit of analysis. Moreover, these studies were performed in few selected single centers.
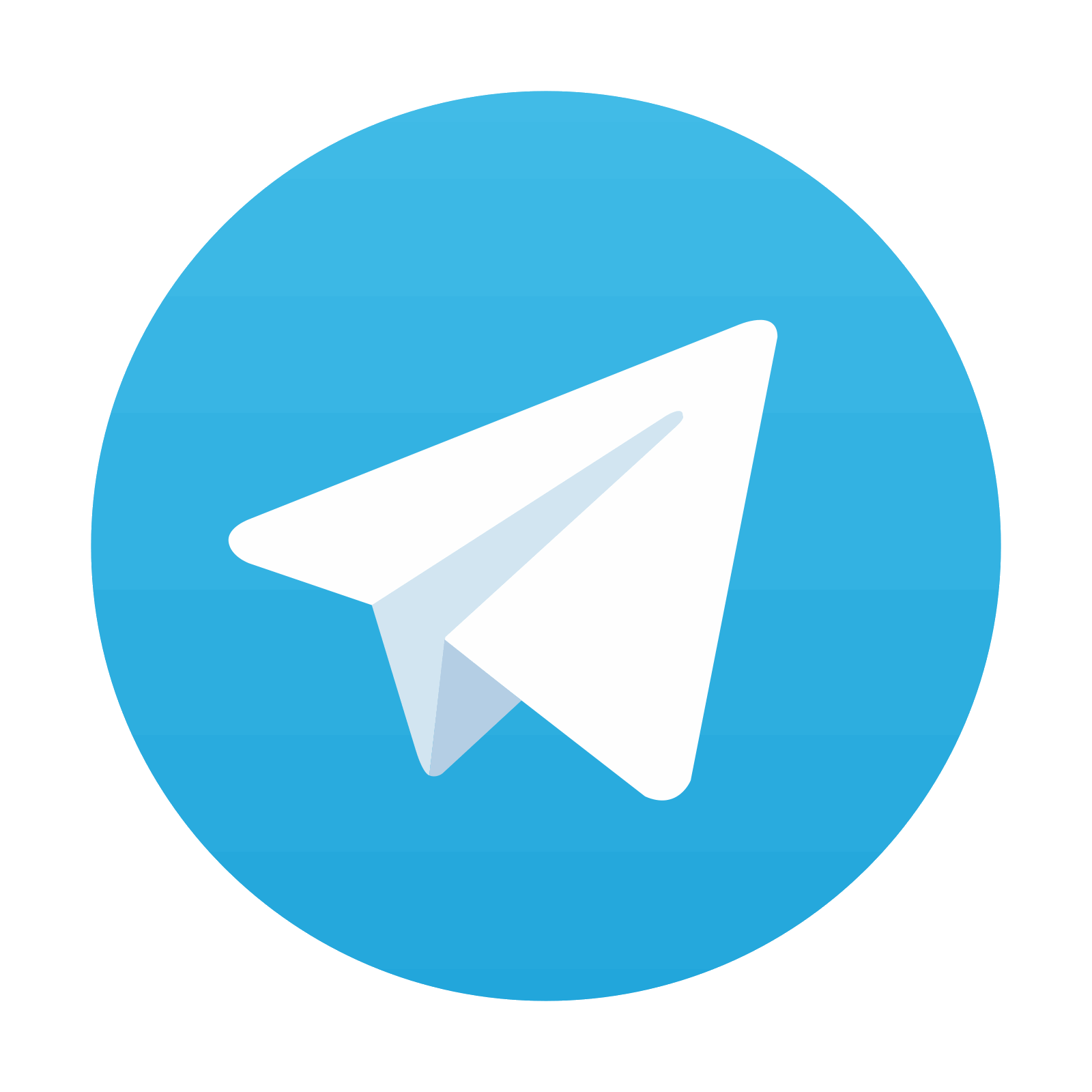
Stay updated, free articles. Join our Telegram channel
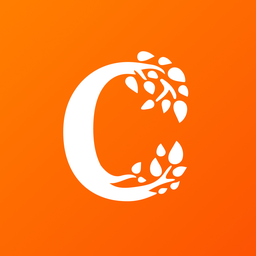
Full access? Get Clinical Tree
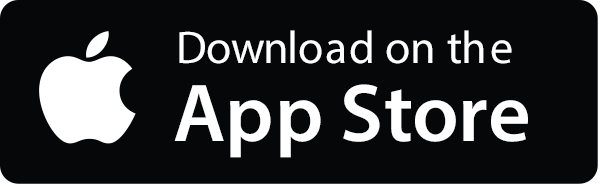
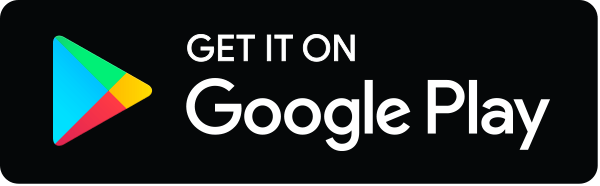