Fig. 7.1
Color duplex ultrasound image of an internal carotid artery showing calcified plaque where a very dense acoustic signal is registered (arrow) with acoustic shadowing (underneath the arrrow)
The ultrasonic carotid plaque morphology may correlate qualitatively with its histological composition; however, the clinical relevance of this information is somewhat controversial [6–9]. The B-mode characteristic of the carotid plaque that appears to correlate most closely with the clinical outcome is heterogeneity. This is generally defined as a plaque that has a mixture of hyperechoic, hypoechoic, and isoechoic plaques, a feature that may be attributed to the presence of intraplaque hemorrhage. This feature has been noted more frequently in patients with neurological events than in asymptomatic carotid stenoses.
Doppler Spectral Waveform Analysis
A valuable adjunct to B-mode imaging is the use of spectral analysis to analyze the backscattered Doppler signal. Spectral analysis, as applied to Doppler ultrasound, is merely a method of determining the frequency content of the backscattered signal and the relative strengths or amplitude of these component frequencies. The original technique was utilized offline and employed a Kay sonograph, which, although providing more information than was previously available with analog displays, was time-consuming and did not depict forward and reverse flow. Real-time spectral analysis was introduced using fast Fourier transform (FFT) analysis. The FFT method makes it possible to display the individual frequencies that make up the return signal. Information related to the intensity of the spectrum is also possible, for example, a narrow well-defined spectrum is displayed when a limited number of frequencies are evident in a laminar flow. Spectral broadening represents a variety of frequencies and is often associated with turbulent flow. The velocity profile shows various frequency shifts on the vertical axis and time on the horizontal axis. The FFT has the advantages of saving time and detecting both forward and reverse flow. This method of signal processing was particularly suitable because pulsed Doppler beams were being utilized in the echo component. Other techniques of spectral analysis, including multiple band pass filter analysis and time compression analysis, have been used, but have not achieved widespread acceptance.
The availability of the pulsed Doppler technique made it possible to obtain velocity information from a known location and, depending on the sample volume size, from a finite volume of the flow stream. The continuous-wave (CW) instruments utilized widely at that time for the detection of disease in the lower extremity were known to have a large sample volume that traversed the whole width of the vessel being insonated and were really most suitable for determining the mean velocity in the forward or reverse direction. Also, the data analyzed by CW instruments were obtained not only from the vessel of interest but also from other vessels in close proximity. With the pulsed instrument, the examiner could be certain that the data were being obtained from the vessel of interest. It was also possible, because of the finite sample volume, to interrogate a segment of the velocity profile and, perhaps, to detect changes that would not otherwise be apparent with a CW device.
A series of animal studies were conducted to determine the relationship between varying degrees of stenosis and spectral changes as identified by pulsed Doppler and fast Fourier spectrum analysis [10].
The Doppler spectral waveform analysis is a signal processing technique that displays the complete frequency and amplitude content of the Doppler signal. This Doppler-shifted frequency is directly proportional to blood cell velocity, and the amplitude of the Doppler signal depends on the number of cells moving through the pulsed Doppler sample volume. The signal amplitude becomes stronger as the number of cells producing Doppler frequency shift increases. This spectral information is usually presented graphically with time on the horizontal axis and frequency or velocity on the vertical axis; and amplitude is indicated by shades of gray (Fig. 7.2).


Fig. 7.2
A normal internal carotid artery Doppler spectra (see bottom of picture)
The following is an explanation of these findings as it applies to the flow patterns within vessels. The center stream flow pattern in a normal artery is uniform or laminar, and a spectral waveform taken with the pulsed Doppler sample volume in the center of the lumen shows a relatively narrow band of frequency. It appears that even relatively mild degrees of stenosis are capable of producing deviations from laminar flow (as zones of vorticeal shedding) in the area distal to the stenosis (Fig. 7.3), with the magnitude of these disturbances being depicted by the magnitude of change in spectral width (spectral broadening). With hemodynamically significant stenoses, not only is spectral broadening present, which is produced by a major decay in the laminar flow pattern, but there is also a marked elevation in peak frequency or peak velocity at systole as a result of the high-speed jet of blood passing through and immediately beyond the stenosis (Fig. 7.4). High-grade stenoses can, therefore, be recognized by the presence of both elevations in peak frequency/velocity at systole and diffuse spectral broadening [5]. The end diastolic frequency or velocity is also increased in very severe stenoses. The Doppler spectral waveform criteria for classifying severity of carotid artery stenosis will be described in detail later.



Fig. 7.3
Schematic representation of minor flow disturbance generated by nonhemodynamically significant stenosis with production of vorticeal shedding immediately beyond the area of the stenosis with resumption of a normal laminar flow pattern further downstream

Fig. 7.4
Schematic representation of major flow disturbance produced by hemodynamically significant stenosis with both increases in peak velocity in and immediately beyond the stenosis with decay of laminar flow to turbulent flow occurring at a maximum two diameters distal to the stenosis
The current application of duplex scanning in the detection of carotid artery disease utilizes this principle of identification of flow disturbance patterns by Doppler velocity detection instrumentation, with the emphasis in later years being on technical improvements in instrument design. A variety of duplex scanning instruments are available, the major differences among them being in the Doppler component. These are of two types: those utilizing CW Doppler and those utilizing pulsed Doppler beams. The outline below applies to the instrumentation available that currently uses pulsed Doppler beams for velocity detection and image generation.
Duplex Instrumentation
The most significant changes in duplex instrumentation have occurred in scan head design. The shape of the pulsed Doppler beam, and therefore its sample volume, has been modified using either medium-focus or short-focus scan heads. The medium-focus scan head, operating at 5 MHz, has a 40-mm focal point, while the short-focus scan head, at a transmitting frequency of 5MHz, has a 20-mm focal point. The beam width of the medium-focus scan head is most narrow at 35–45mm depth, whereas that of the short-focus scan head is most narrow at 20–30 mm. The medium-focus scan head is, therefore, more appropriate for evaluating blood flow in vessels deeper than 30 mm, while the short-focus scan head is ideal for evaluating flow in vessels located close to the surface, 2–3 cm from the skin. Because the carotid arteries lie within 30 mm of the skin surface in the majority of human subjects, the short-focus scan head, at least theoretically, is ideal for evaluating these vessels.
These features are not only important in a consideration of the depths of the vessels studied but also in understanding the effects of the sample volume size on the velocity profile being evaluated. If a large sample volume size is used in the evaluation of small-diameter vessels, a wide range of velocities will be detected under normal circumstances, which on spectral analysis will appear as spectral broadening. In these circumstances, this finding is normal and is similar to the spectra generated by CW instruments. Conversely, if a small sample volume is used in a large vessel, particularly if flow is axisymmetric, the velocities in the sample volume are likely to be similar and, on spectral analysis, will not display spectral broadening. At a range of 25 mm, the beam widths for the medium- and short-focus scan heads are 5.5 mm and 2 mm, respectively, at the 20 dB level. At this range, the sample volumes have been calculated at 3 and 24 mm3 for the short- and medium-focus scan heads, respectively. If spectral broadening, therefore, is an important feature in the evaluation, it is apparent that a short-focus scan head should be more sensitive than a medium-focus scan head.
An additional feature of the current instruments is the dedicated use of the pulsed signal to the Doppler component, which avoids the problem of aliasing encountered in the original prototypes. In the latter, the signal was shared between the echo and Doppler components and resulted in a limited peak frequency detection capability that could be exceeded when severe disease was present. With the pulsed echo component nonoperative, the usual pulse repetition frequency available to the Doppler component is doubled, increasing the frequency response of the 5 MHz instrument at 60° to 9.5 kHz, which is more than adequate to detect the frequencies associated with severe disease.
The quadrature outputs of the pulsed Doppler signal are then analyzed using an online fast Fourier transform spectral analyzer, providing a full-scale frequency display of 10 kHz, with 7 kHz usually being used for forward frequencies and 3 kHz for reverse frequencies. The amplitude of the component frequencies in the signal is depicted in gray-scale format on the oscilloscope screen.
To improve the signal-to-noise ratio on the spectral display, the signal in many instruments is “normalized,” a principle that increases the highest amplitude of each analysis in the spectrum to a particular reference level with the subsequent same scaling factor being applied to all other amplitudes. Following this normalizing process, a variable amount of signal is then displayed depending on the dynamic range used with the Doppler signal. The use of a wide dynamic range enhances the likelihood that in addition to the Doppler backscattered signal, noise will also be displayed. Narrow dynamic range is ideal for evaluating the Doppler signal only.
The addition of high-definition imaging (HDI) technology revolutionized the front end of the ultrasound image formation process. The extended signal processing, or ESP technology (Advanced Technology Laboratories/Phillips system), extends the momentum into the area of signal processing. The result is a substantial reduction in speckle noise, allowing a higher level of clarity and detail than has ever been seen in ultrasound images. Tissue differentiation and resolution of fine anatomical detail, already hallmarks of HDI images, are enhanced even further through the addition of ESP technology.
The technology developments that make HDI and extended signal processing possible are many and complex. Perhaps the most appropriate place to begin is with the acoustic information that is returned to the ultrasound system from the body.
Each tissue within the body responds to ultrasound energy of different frequencies in a characteristic way, which is often referred to as the tissue signature. The tissue signature information is carried within the spectrum of ultrasound frequencies returning from the tissue. This band of frequencies is referred to as the frequency spectrum bandwidth, or, simply, bandwidth.
HDI preserves the quantity and quality of tissue signature through the capture and preservation of the entire bandwidth. This results in more sonographic information with better detail and definition.
The ultrasound beam former, together with the scan head, determines the ultimate contrast resolution, spatial resolution, penetration, and consistency of the image. If the acoustic information containing the tissue signature is reduced in quantity, or distorted in the beam former, there is no way of recovering it. Beam formation is accomplished by pulsing the transducer elements in the scan head to insonify the target. Sound waves reflected by the target return to the elements of the transducer, generating signals that are essentially separated in time. The beam former delays these signals so when all the channels are summed together, the time variations in the signals are compensated for, and the exact tissue definition is obtained. The critical design requirements of the beam former are to preserve the entire bandwidth, which contains all of the acoustic information and to prevent distortion of the signal during delay.
Later, SonoCT real-time compound imaging was incorporated into duplex technology. Using up to nine “lines of sight,” SonoCT imaging dramatically enhances image quality by providing up to nine times more information than conventional two-dimensional imaging. The resulting real-time image is a more realistic representation of actual tissue.
The clinical benefits of SonoCT real-time compound imaging include improved visualization of plaque border delineation, better assessment of plaque morphology, reduction of clutter artifacts seen in difficult-to-image patients, and reduction of posterior plaque shadowing to reveal the full extent of vascular disease. An example of this system is the Model iU22 xMATRIX (Philips Healthcare, Bothell, WA) which has a breakthrough processing technology that optimizes image quality down to the pixel level. It displays a SonoCT image with unprecedented visualization of tissue texture, borders, and margins, almost free of image-degrading artifacts (Fig. 7.5).


Fig. 7.5
A SonoCT image showing tissue texture, borders, and margins
Carotid Duplex Examination Technique
The technician can use any duplex ultrasound imaging system, which includes high-resolution B-mode imaging, pulsed Doppler, and a frequency spectrum analyzer. The carotid examination can be done using several scan heads, e.g., mechanical sector , phased array, or linear array . A linear array scan head is generally used for the mid/distal common carotid artery and carotid bifurcation where these vessels are usually parallel to the skin surface. Meanwhile, the sector-type imaging of a curved array or the smaller footprint phase array may be needed in an examination of the distal internal carotid artery and the vessels in the supraclavicular area.
The examination is conducted with the patient supine and the head slightly extended and turned slightly away from the side being examined and supported to eliminate lateral movement. Copious quantities of water-soluble acoustic gel are applied along the anterior border of the sternomastoid muscle, and the scan head is applied to the skin surface. A 7.5 or 5 MHz transducer is usually used. Presently, we are using the iU22 xMATRIX system, Philips, Bothell, WA (Fig. 7.6). If color flow imaging is used, Doppler information is displayed on the image after it is evaluated for its phase (i.e., direction toward or away from the transducer) and its frequency content (i.e., a hue or shade of color). The sample volume of the pulsed Doppler should be kept as small as possible and placed in the center of the vessel or the flow channel. A Doppler angle of 45–60° should be maintained to obtain consistent results in velocity measurements. The vessels are examined both in longitudinal (Fig. 7.7) and transverse views (Fig. 7.8) and followed from the clavicle to the mandible with anterior oblique, lateral, and posterior oblique projections to identify and evaluate any carotid plaques or pathology.




Fig. 7.6
A duplex ultrasound machine—Model iU22 xMATRIX, Philips Healthcare, Bothell, WA

Fig. 7.7
(a) Gray scale of right common carotid artery bifurcation in longitudinal view. (b) Color duplex ultrasound of right common carotid artery bifurcation in longitudinal view. (c) Power Doppler image of right common carotid artery bifurcation in longitudinal view

Fig. 7.8
(a) Common carotid artery bifurcation in transverse view (gray scale). (b) Common carotid artery bifurcation in transverse view (color flow)
Most of the carotid duplex examination is done using the longitudinal view since it allows the most favorable Doppler angle for recording the velocity data and for color Doppler imaging. Neither the color Doppler image nor the B-mode image will reliably pinpoint the area of maximum velocities and/or flow disturbances, i.e., B-mode imaging and color Doppler are generally helpful for localizing a specific area of interest, which needs to have extra or specific interrogation with pulsed Doppler sampling. Therefore, the gray-scale and color Doppler imaging alerts the technician to the presence of pathology/plaque in the arterial wall that may preclude penetration of the vessel by the pulsed Doppler. It should also be noted that velocity waveforms should not be obtained from a transverse view since the Doppler angle in such a view is either unknown or close to being perpendicular to the flow direction, which will result in useless Doppler velocity measurements. Similarly, color Doppler imaging in cross-section can be misleading for the same reasons. It should also be noted that ultrasound beam angles for optimal B-mode imaging are different from those required for obtaining Doppler spectral waveforms. As noted in the ultrasound physics chapter, the maximum ultrasound reflection is obtained when the ultrasound beam is perpendicular to the imaged surface. The optimal B-mode imaging angle is, therefore, 90 degrees to the surface of the artery. The angle that yields the highest Doppler frequency shift is zero degrees or parallel to the direction of flow. However, since it is difficult to achieve Doppler angle parallel to the flow in the carotid arteries in the neck, an angle of up to 60 degrees between these vessels and the Doppler beam is acceptable. Angles of greater than 60 degrees will produce erroneous higher velocities; meanwhile, angles much lower than 60 degrees may reduce aliasing. The technician may observe changes in the hue of the color flow pattern or bleeding of the color outside of the vessel wall (color bruit, Fig. 7.9), which suggests the presence of stenosis.


Fig. 7.9
Color duplex ultrasound of the internal carotid artery : color bruit
The scan head is then moved cephalad with the B-mode imaging display activated, and with frequent sampling of the center stream velocity signal. Audible interpretation alone is usually used during this phase of the examination. The region of the carotid bifurcation is identified by the presence of two vessels and visualization of the superior thyroid artery branch of the external carotid artery. This may be confirmed by sampling in the center stream just distal to the origin of these vessels and identifying the characteristic differences between the two arteries. It is recommended that the dynamic range be set to 40–50 dB to optimize the gray-scale image and the time gain compensation (TGC) as needed, in regard to the depth of the carotid and vertebral arteries examined.
The external carotid signal is recognized by the presence of flow reversal , while the internal carotid signal is identified by the absence of flow reversal and the presence of forward flow during diastole. The scan head is moved further cephalad to insonate the proximal few centimeters of the internal carotid artery, which is the common site of disease. Abnormalities in the velocity spectra displayed on the screen are noted for subsequent reference. Once the general anatomy has been outlined, a detailed examination is performed. The initial quick scanning of the vessels provides a reference for determining whether disease is present and, if so, its severity. It is likely that these areas will require more detailed interrogation than areas that are normal.
Following the preliminary scan, the scan head is returned to the base of the neck over the anterior border of the sternomastoid muscle, and the common carotid artery is again visualized. Note is taken of the presence or absence of calcification in the wall represented by dense acoustic shadows and a deeper acoustic window. Representative spectra are then obtained from the center stream with the Doppler beam axis at 60° and the signals recorded for subsequent analysis. During this part of the examination, the peak velocity should be noted and whether the velocity is always in the forward direction throughout the whole of the cycle.
Low peak systolic velocities suggest occlusions of the internal or external carotid arteries, while frequencies approaching zero are suggestive of either high-grade stenosis or occlusion of the internal carotid artery. Other variations in the waveform may occur as a result of significant aortic disease.
The scan head is again moved cephalad with a second center stream sample being obtained just proximal to the region of the bulb. With rapid shifting from B-mode to Doppler mode imaging, the evaluation is continued through and into the proximal internal carotid artery, looking for abnormal spectral displays. Care must always be taken during sampling to ensure that the sample volume cursor is located in the center stream of the vessel and the incident angle of the Doppler beam to the long axis of the vessel is as close as possible to 60°. The presence of disease is suspected by echogenic shadows impinging on the lumen of the vessel associated with either changes in spectral broadening or fluctuations in peak systolic and diastolic velocities. It is frequently necessary to obtain multiple spectra along the center stream axis of the internal carotid artery to determine the location at which the most abnormal spectra occur. These should be recorded for future reference.
Attention is then directed to the subclavian artery in the posterior triangle of the neck, and the vessel is visualized. Scanning proceeds proximally with identification of the origin of the vertebral artery and subsequent sampling with the Doppler component of the orifice in the proximal centimeter of the first portion of this vessel, as this is the usual site of stenotic disease.
With a clear view of the common carotid artery, the probe is slowly angled more posterior-laterally to identify the vertebral artery. This artery will have vertical shadows running through it from the spinous processes of the vertebrae, giving it the appearance of a series of H’s (Fig. 7.10). Vertebral flow is documented, either antegrade or retrograde. Major elevations in peak velocities are characteristic of high-grade orifice stenosis. The contralateral side of the neck is then evaluated in a similar manner, and representative recordings from the common carotid, external carotid, and internal carotid arteries are obtained.


Fig. 7.10
(a) Origin of right vertebral artery in gray scale. (b) Origin of right vertebral artery using color duplex ultrasound. (c) Origin of right vertebral artery using power Doppler. (d) Mid right vertebral artery (series of H appearance)
The following considerations are generally helpful in optimizing color flow setup and value. The appropriate color pulse repetition frequency (PRF) must be chosen by setting the color velocity scale for the expected velocities in the examined vessel. The scale should be adjusted to avoid systolic aliasing (low PRF) or diastolic flow gaps (high PRF) in normal vessels. Every effort should be made to avoid using large wider color boxes, which may slow down frame rates and resolution of the imaged vessel. It is recommended that color boxes that cover the entire vessel diameter and are approximately 1–2 cm of its length be used. The color, power, and gain should be optimized so that flow signals are recorded throughout the lumen of the examined vessel with no bleeding of color into the adjacent tissues.
The zero baseline of color bar (PRF) is set at approximately two-thirds of the range with the majority of frequencies allowed in the red direction for flow toward the brain, which will display higher arterial mean frequency shifts without aliasing artifacts. The color PRF and zero baseline may also need to be readjusted throughout the examination to allow for changes in velocity that may occur if carotid tortuosity or stenosis is present. Adjustments in the PRF are generally needed in the examination of the carotid bulb where the color differentiation scale should be set to visualize the slower flow in the boundary separation zone (Fig. 7.11). The PRF range is generally adjusted higher to detect increased velocity in the region adjacent to the flow divider. Similarly, the color PRF should be increased to display higher velocities detected in the presence of carotid stenosis and to avoid aliasing. In the post-stenotic zone, the color PRF should be decreased to observe the lower velocities and flow direction changes in the region of turbulent flow just distal to the stenosis. Color PRF should also be decreased when occlusion is suspected to detect the preocclusive, low velocity, high resistant signal associated with tight stenosis or carotid occlusion and to confirm absence of flow at the site of the occlusion. The color PRF should also be decreased in the presence of a carotid bruit to detect the lower frequencies associated with a bruit.


Fig. 7.11
Color duplex ultrasound of the internal carotid artery—notice boundary separation zone (blue color)
Color sensitivity (ensemble length) should be around 12 in systems where there is an adjustable control. The ensemble length can be increased in regions where more sensitive color representation is needed. Keep in mind that the frame rate will decrease when the ensemble length is increased. The color wall filter should also be set as low as possible, and you may need to decrease the wall filter manually when decreasing the color PRF. The color wall filter may automatically increase as the PRF is increased.
The angle of the color box should also be changed to obtain the most accurate Doppler angle between the scan lines and the direction of the blood flow. This will yield a better color display, secondary to better Doppler angle. The color box should be kept to a size that is adequate for visualizing the area of interest and should be kept small enough to keep the frame rate at a reasonable number. The color gain should be adjusted throughout the examination to detect the changing signal strength. If this is not properly adjusted, too much color may be displaced, or some color information may be lost, which may result in seeing color in areas where there is no flow. In patients with very low flow or questionable carotid occlusion, an over-gained level may be advantageous to show any flow that may be present.
The desaturation of the color from darker to lighter hues on the color bar indicates increasing velocities. The colors are darkest close to the zero baseline, and as the velocities increase, the colors become lighter. Color should be selected so that the highest frequency shifts in each direction are of high contrast to each other so that you can easily detect aliasing, e.g., the color selection can be set so that low to high velocities are seen as dark blue to light green to aqua in one direction and red to orange to yellow in the opposite direction. Aliasing in these circumstances would appear as aqua, adjacent to yellow.
Since the frame rate is affected by the PRF, ensemble length, depth, and width of the color box, it should be kept as high as possible to capture the rapid change in flow dynamics that occurs with carotid stenosis, particularly in the carotid bulb region. The frame rate decreases with decreasing PRF, and increasing the color ensemble length will also decrease the frame rate. Increased color box width and deep insonation will also decrease the frame rate.
Limitations of Duplex Technology
Duplex technology of the carotid arteries may be adversely affected by the following: acoustic shadowings from calcification, soft tissue edema or hematoma, the depth or course of the vessel, the size of the neck, and the presence of sutures or skin staples.
Duplex ultrasonography may also overestimate or underestimate the degree of stenoses or plaquing. Underestimation of disease can be noted if it fails to appreciate very low level echoes of soft plaque; or the examiner does not carefully interrogate the vessel and misses accelerated flow; or in patients with long, smooth plaque formation, which does not have the accelerated, turbulent flow pattern usually associated with the hemodynamically significant stenoses; or if an inappropriate Doppler angle is used (e.g., above 60°). Stenoses can also be overestimated when an artifact is mistaken for a carotid plaque, if accelerated flow is mistakenly attributed to stenosis, if there is vessel tortuosity or kinking, and in the presence of significant stenoses or occlusion on the contralateral side.
Due to the varying filling phases of the cardiac cycle, cardiac arrhythmia makes it more difficult to evaluate the flow spectra. Also, the flow velocity will be lower in a wider vessel and higher in a narrower vessel at the same flow intensity. Therefore, the flow in a wide carotid sinus can easily be disturbed and may incorrectly suggest pathological findings.
Interpretation and Determination of Severity of Carotid Stenosis
A complete extracranial carotid duplex examination should include the following data:
- 1.
The peak systolic and end diastolic velocities of common carotid, internal carotid, and external carotid arteries, right and left subclavian arteries, and vertebral arteries
- 2.
The internal carotid artery to common carotid artery peak systolic velocities ratio
- 3.
Flow direction of the vertebral artery (antegrade or retrograde)
- 4.
Analysis of the Doppler spectral waveform of the examined vessels
- 5.
The presence or absence of plaque and description of its morphology
B-Mode Imaging Interpretation
An echoic area should be evident between the walls of the vessel, indicating the absence of pathology, i.e., plaquing, whose density usually differs from that of the blood. An echoic line indicating the endothelium may be evident at the vessel lumen. The following abnormalities can be noted on B-mode imaging:



- 1.
Fatty streaks and low level echoes of similar appearance (homogeneous) can be detected.
- 2.
Fibrous soft plaque (homogeneous): low to medium level echoes of similar appearance (Fig. 7.12).
- 3.
Complex plaque (heterogeneous): low, medium, and high level echoes indicating soft and dense areas (Fig. 7.13). This plaque is a mixture of isoechoic, hyperechoic, or hypoechoic plaque.
- 4.
Calcification: very bright, highly reflected echoes are noted. The acoustic shadowing from calcifications prevents a thorough evaluation of the vessel and may result in the calculation of an erroneous percentage of stenosis (Fig. 7.14).
- 5.
Vessel thrombosis: fresh carotid thrombosis may not be detected without using Doppler flow sampling since fresh thrombus has the same echogenicity of flowing blood.

Fig. 7.12
B-mode image of the left distal common carotid artery showing a calcified smooth plaque (arrows)

Fig. 7.13
(a) Color duplex ultrasound image of the carotid bifurcation showing a complex heterogenous plaque at the origin of the internal carotid artery (arrow). (b) A duplex ultrasound image of the carotid artery showing a heterogeneous plaque (arrow)

Fig. 7.14
A duplex ultrasound image of the carotid artery showing a calcified plaque with acoustic shadowing underneath (arrow)
Carotid plaque morphology is generally characterized into smooth (Fig. 7.15) or irregular plaques (Fig. 7.16) according to surface and homogeneous (Fig. 7.12) versus heterogeneous (Fig. 7.13) according to plaque structure. An ulcerative plaque is usually an irregular plaque with a cleft within the plaque that can be seen on B-mode imaging (Fig. 7.17). Further details of carotid plaque morphology will be described in Chap. 8.




Fig. 7.15
A color duplex ultrasound image of the carotid artery showing a smooth heterogeneous plaque (arrow). The dark center of the plaque may represent intraplaque hemorrhage

Fig. 7.16
A duplex ultrasound image showing an irregular plaque of the proximal right internal carotid artery (arrow)

Fig. 7.17
A duplex ultrasound image of the carotid bifurcation showing an ulcerative lesion of the proximal right internal carotid artery (arrows)
Estimation of Stenosis Based on B-Mode Imaging
Ideally, carotid plaque should be visible from at least two of the longitudinal, or sagittal, projections and in the transverse view to give a rough estimate of stenosis. Percent diameter stenosis equals the ratio of the residual diameter to vessel lumen diameter minus 1 multiplied by 100. Percentage of area of stenosis is calculated similarly, except you substitute the area for the diameter. The vessel lumen diameter (in longitudinal view) or area (in transverse view) is measured from intima to intima. Then the residual lumen diameter, or area, is measured (Fig. 7.18). The percent reduction is calculated using the above formula. The approximate relationship between diameter and area of stenosis is shown in Table 7.1. These values are applicable to circular geometry.


Fig. 7.18
Calculation of area reduction percent stenosis . This image reflects the greatest stenosis in transverse diameter. An elliptical measurement of the arterial lumen is taken. Then an elliptical trace of the residual lumen is made. The percent area reduction is calculated by the duplex machine. To calculate the diameter reduction percent stenosis, a similar calculation is performed with the vessel in longitudinal view
Table 7.1
% Diameter stenosis vs. % area stenosisa
Diameter stenosis | Area reduction |
---|---|
% Stenosis by | |
0 | 0 |
10 | 19 |
11 | 36 |
30 | 51 |
40 | 64 |
50 | 75 |
60 | 84 |
70 | 91 |
80 | 96 |
90 | 99 |
100 | 100 |
A chronic arterial occlusion may be diagnosed using B-mode imaging, although Doppler interrogation is essential to this diagnosis. Depending on the type of occlusive process, the artery may be filled with highly echogenic material or be anechoic.
Interpretation of Doppler Spectral Analysis
Normal Findings
The external carotid artery supplies blood to the vascular bed that has high peripheral resistance. Therefore, its signal is more pulsatile and very similar to the signal from peripheral arteries, such as the common femoral artery. As shown in Fig. 7.19 the external carotid artery has a rapid upstroke and downstroke with a very low diastolic component. The diastolic notch is clearly seen, and tapping the superficial temporal artery causes oscillations in the waveform (Fig. 7.19).


Fig. 7.19
Color duplex ultrasound image of an external carotid artery . Note that the external carotid artery has a rapid upstroke and downstroke with a very low diastolic component. The diastolic notch is clearly seen, and tapping of the superficial temporal artery causes oscillations in the waveform (bottom right)
The internal carotid artery signal is slightly more high-pitched and continuous than the signal from the external carotid artery. The blood flow in the internal carotid artery is less pulsatile since the brain is a low-resistance vascular bed, with increased flow during diastole. As shown in Fig. 7.20, the waveform of the internal carotid artery has a rapid upstroke and downstroke with a high diastolic component. A diastolic notch may not be evident. The common carotid artery, meanwhile, has a flow characteristic of both the internal and external carotid arteries (Fig. 7.21). Since most of the flow of the common carotid artery (close to 80%) enters the internal carotid artery, which has low-resistance flow, the flow in the common carotid artery usually shows a low-resistance pattern with a rapid systolic upstroke and forward flow through diastole.



Fig. 7.20
Color duplex ultrasound image of the internal carotid artery. Note that the waveform of the internal carotid artery has a rapid upstroke and downstroke with a high diastolic component. The diastolic notch may not be evident

Fig. 7.21
A color duplex ultrasound image of the common carotid artery. The common carotid artery signal has a flow characteristic of both the internal and external carotid arteries
Spectral waveforms must be obtained from the proximal (bulb), mid, and distal cervical internal carotid artery segments. The normal proximal ICA (the bulb), which is generally larger than the mid/distal ICA, will have characteristic flow. Unidirectional flow patterns are normally found in the carotid bulb, along the flow divider of the carotid bifurcation, and because there is transient reversal of flow at peak systole near the center stream and at the outer wall opposite to the flow divider, this area of reverse flow is often seen in color Doppler and commonly called an area of boundary layer separation or flow separation. Flow velocities along the outer wall and the separation zone may drop to zero at the end of the diastole. This normal flow pattern is used in conjunction with the absence of visible plaque on B-mode imaging to indicate a normal carotid bulb.
In a pulsed Doppler tracing , and because the sample volume would be more precisely placed in a center stream, the signals will have a narrow band of frequencies in systole with a blank area under that narrow band. The narrow band is called the spectral envelope; the blank area is called the frequency window or spectral window. The presence of these features is generally seen in laminar flow (Fig. 7.22). In contrast, in continuous-wave Doppler, because of the inability to regulate sample size or depth, a frequency window is not clear (Fig. 7.23).



Fig. 7.22
Spectral analysis of pulsed Doppler waveform : (1) spectral envelope, (2) peak systole, (3) frequency window, (4) dicrotic notch, (5) diastole

Fig. 7.23
Left: continuous-wave Doppler signal. Note the absence of a frequency window. Right: in contrast the pulse wave Doppler signal has a frequency window
Abnormal Findings
The auditory signal from a stenotic vessel is characterized by a higher than normal pitch, with a very high-pitched hissing or squealing type of signal evident at significant stenosis. The waveform from a stenotic vessel has a higher than normal amplitude in systole and diastole because of the accelerated flow through the stenosis (Fig. 7.24). In a spectral analysis, the band evident along the top of the waveform during systole may fill in the spectral window to create the spectral broadening that is consistent with turbulent flow. As seen in Fig. 7.24, the more significant the stenosis, the greater the increase in systolic and diastolic frequencies/velocities. In severe stenoses there will be complete loss of the window. Distal to a stenosis, disturbed flow patterns are evident, i.e., dampened monophasic flow (turbulence). Immediately proximal to severe stenosis, the spectral waveform is dampened, and velocities are usually decreased with increased velocities within the stenosis and remain elevated with turbulent flow, which is reflected as spectral broadening just distal to the stenosis. It should be noted that an absent signal may suggest occlusion; however, a tight stenosis cannot be ruled out since blood flow may be difficult to detect with velocities of less than 6 cm/s.


Fig. 7.24
Waveforms from a normal vessel (a) in contrast to a mildly stenotic vessel (b) and a severely stenotic vessel (c). See text for a more detailed description
Occlusion of the internal carotid artery (Fig. 7.25) is usually associated with a loss of the diastolic component in the ipsilateral common carotid artery. If the contralateral common carotid and internal carotid arteries are serving as collateral pathways, increased systolic and diastolic velocities may be evident in these arteries. If a carotid siphon stenosis is present, high-resistance flow patterns may be evident in the extracranial internal carotid artery. Flow characteristics from one side must be compared with those on the other, as well as those in proximal to distal segments of the ipsilateral carotid system. Generally, this test is somewhat limited in patients with poor cardiac output or stroke volume, which may result in bilaterally diminished common carotid artery velocities. Unilateral reduction of velocities may suggest proximal disease, such as innominate or proximal common carotid artery stenoses.


Fig. 7.25
A color duplex ultrasound image of a patient with occlusion of the right internal carotid artery, which is usually associated with a loss of the diastolic component in the ipsilateral common carotid artery (bottom)
Determination of Disease Severity Using Doppler Spectral Analysis
Identification of disease in the carotid system uses both qualitative and quantitative data. Careful attention to unusual echoes on the image serves as a qualitative guide to the presence of disease at sites where careful scrutiny with a Doppler component should be performed. The changes in spectra obtained from the common, internal, and external carotid arteries provide quantitative information for the determination of the severity of disease in these locations. This is probably best considered by describing the normal and abnormal spectra generated in various anatomical locations by disease of varying severity, according to the University of Washington criteria [11]. These original criteria by the University of Washington are described in the following sections (Table 7.2) since they are still used in many vascular laboratories in the United States, and they have been the foundation for interpretation of carotid artery stenosis. However, later in this chapter you will find that other authorities modified these criteria to be compatible with the indication for carotid endarterectomy as proposed by the North American Symptomatic Carotid Endarterectomy Trial (NASCET) and Asymptomatic Carotid Atherosclerosis Study (ACAS) trial.
Table 7.2
Strandness criteria
Stenosis/occlusion | |
Normal | PSV < 125 c/s |
No spectral broadening | |
1–15% stenosis | PSV < 125 c/s |
Minimal spectral broadening | |
16–49% stenosis | PSV < 125 c/s |
Marked spectral broadening | |
50–79% stenosis | PSV ≥ 125 c/s |
EDV < 140 c/s | |
Marked spectral broadening | |
80–99% stenosis | PSV ≥ 125 c/s |
EDV ≥140 c/s | |
Post-stenotic turbulence | |
Occlusion | No flow |
University of Washington Carotid Duplex Criteria (Strandness)
Normal Internal Carotid Spectra or Minimal Disease (0–15% Stenosis)
The characteristic features of normal internal carotid artery spectra are shown in Fig. 7.2. The peak systolic velocity is <125 cm/s, with minimal degrees of spectral broadening during the initial deceleration phase of systole, followed by mild spectral broadening during diastole. The velocities are always in the forward direction, and, therefore, the frequencies depicted on the scale are always above the zero line. The velocity envelope during systole is relatively narrow and displays a large clear window area under the systolic curve. Correlation with arteriographic findings has supported the view that this type of waveform may also be generated with minimal disease up to 15–20% diameter reduction, and, therefore, identification of this type of waveform confirms the presence of either a normal vessel or one in which only minimal disease is present. Figure 7.26 is a color duplex imaging of a normal common carotid artery, an internal carotid artery, and an external carotid artery.


Fig. 7.26
(a) A color duplex ultrasound image showing a normal common carotid artery with a normal common carotid artery with a normal Doppler spectra (bottom of figure). (b) B-mode image of normal carotid bifurcation showing both external and internal carotid arteries. (c) Color Doppler signal from the left proximal internal carotid artery
Mild Stenosis (16–<50%)
As noted in the discussion regarding findings with animal studies, it is over the range of mild stenosis that spectral broadening changes in both magnitude and timing, and it is the presence of spectral broadening in systole, particularly during the deceleration phase, which is characteristic of the spectra generated by the presence of mild disease. As shown in Fig. 7.27, the peak systolic velocity of <125 cm/s and spectral broadening is also present during diastole, although it may be of greater magnitude than seen in the normal. Again, velocity is always in the forward direction, and therefore the frequencies, even during diastole, are above the zero frequency line.


Fig. 7.27
(a) B-mode image showing mild carotid plaque of the left internal carotid artery. (b) Color Doppler of same lesion with Doppler flow
Moderate to Severe Disease (50–<80% Stenosis)
As the lesion becomes progressively more occlusive (50–<80% diameter reduction), the velocity of the red blood cells traversing the stenosis increases, producing an increase in peak velocity at systole (Fig. 7.28). A peak systolic velocity of >125 cm/s and an end diastolic velocity of <125 cm/s are characteristic of this stenosis.


Fig. 7.28
(a) B-mode image showing plaquing of the proximal internal carotid artery (bulb) (arrow). (b) Same patient showing PSV of 236 cm/s and EDV of 42.4 cm/s, consistent with 50–<80% stenosis (or 50–<70% stenosis, based on consensus criteria)
Tight Stenosis (80–99%)
With the development of high-grade lesions in excess of 80% diameter reduction, the end diastolic velocity increases (a peak systolic velocity of ≥125 cm/s and an end diastolic velocity of ≥125 cm/s) so that the ratio between peak frequency at systole and peak frequency at diastole falls, providing an accurate method of identifying these high-grade lesions. Diffuse spectral broadening is also present during the whole of the cycle, and with these lesions, the diastolic velocity at the lower frequencies approaches zero (Fig. 7.29).


Fig. 7.29
(a) Color duplex ultrasound of proximal internal carotid artery showing a PSV of 573 cm/s and EDV of 189 cm/s, consistent with 80–90% stenosis (or >70–99%, based on consensus criteria). (b) Color duplex ultrasound of mid internal carotid artery showing PSV of 437 cm/s and EDV of 115 cm/s, consistent with 80–99% stenosis
Internal Carotid Occlusion
Occlusion of the internal carotid artery (Fig. 7.25) is recognized by imaging a vessel in the characteristic anatomical location of the internal carotid artery with no detectable Doppler signal. It is important to ensure that the internal carotid artery is being examined, and as part of this evaluation, visualization of the external carotid artery is mandatory. The differentiation between the internal and external carotid arteries is made by visualization of the superior thyroid artery branch. Changes in the real-time spectra produced by compression of the superficial temporal artery that increases the outflow resistance usually result in a decrease in peak systolic frequency. Other features characteristic of occlusion are the presence of frequencies to the zero baseline, or even negative frequencies, indicative of flow reversal obtained from the common carotid artery low in the neck [12]. When the internal carotid artery is occluded, the ipsilateral common carotid artery assumes a velocity pattern similar to that of the external carotid artery, and the external carotid artery may assume flow characteristics of the internal carotid artery, i.e., high diastolic component.
Common carotid artery occlusion can also be diagnosed by color duplex ultrasound. Figure 7.30 shows retrograde flow of the external carotid artery and antegrade flow of the internal carotid artery. Occlusions are periodically missed due to changes in physiologic parameters attendant upon the presence of internal carotid artery occlusions. Figure 7.31 shows the arteriogram and spectra obtained from a patient in whom internal carotid occlusion was missed because the external carotid artery was a major source of collateral blood flow to the middle cerebral artery and, as such, developed the spectral changes characteristic of a high-grade internal carotid stenosis. Errors such as this can be avoided by careful evaluation of the image for the presence of branches originating from the vessel being examined and the change in the velocity profile induced by superficial temporal artery compression.
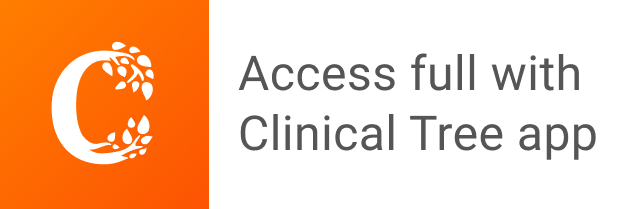