Introduction
A central sleep apnea (CSA) results from a transient abolition of central respiratory drive to the respiratory muscles leading to cessation of airflow; this is most often due to a fall in arterial P co 2 below the threshold required to stimulate breathing. By convention, in adults, apnea is defined as an absence or reduction of airflow to less than 90% of the baseline level for at least 10 seconds. During a central apnea, there is no respiratory effort and therefore no movement of the chest wall; this is in contrast to obstructive apneas, during which central drive and respiratory efforts continue. Hypopneas can also be part of a CSA disorder. In this case, airflow and tidal volume decrease by 50% to 90% compared with normal breathing for at least 10 seconds usually in association with oxygen desaturation or an arousal from sleep, but without evidence of airflow limitation due to upper airway obstruction. Distinguishing central from obstructive hypopneas can be difficult depending on the type of instrumentation used to detect them (see later for more details).
A CSA disorder is defined as recurrent central apneas and hypopneas during sleep. However, a clear threshold above which a CSA disorder can be said to exist has not been well defined owing to its relative rarity and its heterogeneous pathophysiology, clinical symptoms, and complications. Nevertheless, the following criteria—albeit rather arbitrary—have been proposed to define a CSA disorder: an apnea-hypopnea index (AHI) of 5 to 15 (mild), 15 to 30 (moderate), or greater than 30 (severe), of which the majority of events are central. Similarly, criteria for a CSA syndrome have not been clearly defined but include the presence of a CSA disorder accompanied by symptoms, which could include habitual snoring, restless sleep, nocturnal awakenings, morning headaches, insomnia, or excessive daytime sleepiness ( Table 89-1 ).
Characteristic | Hypercapnic | Nonhypercapnic |
---|---|---|
Sex distribution | Equal | Predominantly male |
History of respiratory failure | Frequent | Not reported |
Peripheral edema and cor pulmonale | Frequent | Not reported |
Polycythemia | Frequent | Not reported |
Muscle weakness | Frequent | Not reported |
Morning headaches | Common | Uncommon |
Snoring | Common | Frequent |
Nasal obstruction | Uncommon | Common |
Hypertension | Uncommon | Common |
Nocturnal choking | Uncommon | Common |
Nocturnal awakenings and insomnia | Uncommon | Common |
Daytime sleepiness | Frequent | Frequent |
Restless sleep | Common | Common |
Diagnosis of Central Sleep Apnea
Full overnight polysomnography with instrumentation capable of detecting respiratory effort and airflow limitation is required to diagnose CSA. Respiratory effort is best determined noninvasively by respiratory inductance plethysmography. Techniques such as piezo-electric crystal bands, oronasal thermistors, and measurement of nasal pressure are not reliable in ruling out respiratory efforts and therefore of distinguishing central from obstructive events. Most laboratories do not differentiate hypopneas as being central or obstructive, which is a problem because in patients with OSA or CSA, most respiratory events are hypopneas. Thus, in many cases, the classification of the type of sleep apnea disorder is made on the basis of apneas, which constitute a minority of respiratory events. Central hypopneas are characterized by attenuated in-phase thoraco-abdominal motion due to reduced respiratory drive and in the absence of airflow limitation due to upper airway obstruction. In some cases, more sensitive techniques may be required to detect the presence of respiratory effort or airflow limitation, such as measurement of esophageal pressure or diaphragm electromyographic activity. The use of oxygen desaturation criteria for hypopneas is controversial because central events in patients with heart failure are accompanied by less oxygen desaturation than obstructive events of similar duration, and it is unclear to what extent oxygen desaturation contributes to morbidity or mortality.
Classification of Central Sleep Apnea
CSA is not a single disease entity but rather includes several heterogeneous disorders as outlined in Table 89-2 . Nevertheless, common to all forms of CSA is that arterial P co 2 comes to lie below the level required to stimulate breathing during sleep (i.e., the apnea threshold). The P co 2 can be lower than the apnea threshold because of either a decrease in P co 2 or an increase in the apnea threshold. A CSA caused by an increase in the apnea threshold with onset of sleep is illustrated in Figure 89-1 . On the basis of theoretical, experimental, and clinical considerations, two distinct underlying mechanisms can account for cessation of central respiratory output during sleep.
Hypercapnic (P co 2 > 45) (Decreased respiratory drive) |
|
Nonhypercapnic (P co 2 ≤ 45) (Normal or increased respiratory drive) |
|


First, outright defects in the respiratory control system or in the respiratory neuromuscular apparatus can lead to central apneas. Such defects generally result in suppressed respiratory drive, manifested by some degree of daytime hypercapnia. However, the full impact of such defects becomes most apparent during sleep, when nonchemical waking neural drive to breathing is abolished, and the stimulatory influence of behavioral, cortical, and reticular inputs to the brain stem respiratory neurons are reduced. In this situation, breathing becomes critically dependent on the defective metabolic respiratory control system, resulting in more pronounced hypercapnia during sleep than during wakefulness. In this case, central apnea is triggered by a fall in P co 2 below the apnea threshold but in conjunction with a markedly elevated P co 2 apneic threshold. Figure 89-2 illustrates a typical central apnea in a patient with hypercapnic CSA: As respiratory drive diminishes during sleep, there is a gradual reduction in tidal volume until breathing stops.

Second, transient fluctuations or instabilities in an otherwise intact respiratory control system can lead to central apneas. These instabilities typically arise during drowsiness and during light, non–rapid eye movement (non-REM) sleep and, because there is no suppression of respiratory drive, arterial P co 2 levels during wakefulness or sleep are normal or low. This type of CSA is typically triggered by a sudden increase in ventilation that causes P co 2 to fall below the apnea threshold, often due to an arousal from sleep as illustrated in the upper panel of Figure 89-3 . This type of CSA is characteristically associated with periodic breathing, during which there are regularly recurring alterations in tidal volume between central apneas/hypopneas and hyperpneas. Several theoretical and experimental models have been developed to account for such transient fluctuations in central respiratory drive. Common to all these models is a P co 2 level during sleep that falls transiently close to (hypopnea) or below (apnea) the critical threshold value required for maintenance of regular respiratory rhythm.

Such transient discrepancies in P co 2 levels arise in a number of conditions, the most common of which is the transition from wakefulness to sleep. Because of the waking neural drive to breathe, ventilation is higher and P co 2 levels are lower during wakefulness than during sleep. Withdrawal of this drive at the transition from wakefulness to sleep results in a P co 2 level that, although appropriate for wakefulness, is below the value appropriate for sleep (see Fig. 89-1 ). If this P co 2 level of wakefulness is below the threshold value for rhythm generation during sleep, apnea will ensue at sleep onset until P co 2 rises to the critical threshold value when ventilation will resume. If sleep becomes firmly established at this point, regular breathing resumes without further apneas or hypopneas. However, in some individuals prone to periodic breathing, the transition from wakefulness to sleep is characterized by repeated fluctuations in central nervous system state between “awake” and “asleep.” With each momentary arousal from sleep toward wakefulness, the P co 2 levels that were present during sleep represent a hypercapnic stimulus during wakefulness, and ventilation therefore increases in accordance with the awake response to carbon dioxide, resulting in a hyperpneic phase during which there is ventilatory overshoot causing P co 2 to fall below the apnea threshold on the transition to sleep. In this scheme, the magnitude of fluctuation in ventilation with changes in central nervous system state depends on the difference between the awake and sleeping values of P co 2 and the steepness of the ventilatory response to carbon dioxide during wakefulness. Any factor that magnifies these variables enhances the tendency to periodic breathing and CSA.
Hypercapnic Central Sleep Apnea
The automatic process of breathing originates from the central respiratory rhythm generator located in the pons and medulla. This central generator provides most of the input to spinal motoneurons responsible for activation of respiratory muscles. Hypercapnic CSA results from processes that damage the cerebral cortex, brain stem, spinal cord, muscles, or motor nerves. CSA with hypercapnia almost always presents as chronic respiratory failure with hypercapnia both during wakefulness and sleep due mainly to a reduction in central respiratory drive. At the transition from wakefulness to sleep, the already reduced central drive is further reduced, causing a gradual reduction in ventilation until there is complete cessation of respiratory drive that leads to transient central apneas (see Fig. 89-2 ). Coincident with this reduction in ventilation, P co 2 rises and arterial S o 2 falls. In contrast to nonhypercapnic CSA, apneas and hypopneas are more frequent during REM sleep than non-REM sleep owing to a further reduction in ventilator drive and partial paralysis of the respiratory muscles in REM sleep.
Secondary Forms of Hypercapnic Central Sleep Apnea
Developmental and Degenerative Diseases.
The brain stem includes the medulla, midbrain, and pons. Although the central respiratory controller is located predominantly in the medulla, it has projections to other areas of the brain stem. Diseases affecting the brain stem, such as tumors, stroke, Chiari malformations, and neurodegenerative diseases, may induce hypoventilation and CSA via a number of mechanisms such as (1) physical compression of the brain stem resulting in damage to the respiratory center and reticular activating system, (2) stretching of the lower cranial nerves and damage to the peripheral chemoreceptors that carry inputs from the carotid bodies to the medulla, (3) depletion of medullary chemosensitive respiratory neurons, or (4) degeneration of the central pattern generator located in the dorsolateral pons.
Chiari malformations are congenital abnormalities of the craniocervical junction with downward displacement of the cerebellar structures often in association with syringomyelia, myelomeningocele, hydrocephalus, and aqueductal stenosis. Chiari malformations are classified into three types. Chiari malformation type I is characterized by abnormally shaped cerebellar tonsils with herniation below the foramen magnum that is associated with the Klippel-Feil anomaly and spinal cavitations. Type II is characterized by the downward displacement of the cerebellar vermis and tonsils below the foramen magnum with associated obstruction of cerebrospinal fluid flow and hydrocephalus, in addition to a myelomeningocele. Type III is downward displacement of the medulla with an occipital or cervical encephalocele. These anatomic abnormalities can lead to degeneration of brain stem respiratory neurons with resulting hypoventilation and, in some cases, CSA.
In those with type I Chiari malformation, a prospective study in children showed a prevalence of CSA of 9%, with an increased risk of CSA in those with hydrocephalus. In adults with Chiari malformations (types I and II), CSA prevalence was reported to be 15%. In a small cross-sectional study of patients with Chiari malformation type II and associated myelomeningocele, the prevalence of CSA was much higher: 63%.
Congenital central hypoventilation syndrome (CCHS) is a rare genetic disease with an incidence of approximately 1 in 200,000 live births, caused by a mutation of the PHOX2B gene. The majority of cases present in the neonatal period or in early childhood with acute or chronic respiratory failure as a result of a loss of respiratory drive. However, it may present de novo in adults following a severe respiratory infection with combined central and obstructive sleep apnea (OSA). A diagnosis is considered only in those in whom metabolic, neurologic, pulmonary, and medication-induced disorders have been excluded. When CCHS is suspected, the PHOX2B screening test is recommended for purposes of genetic counseling.
Neurodegenerative diseases of the brain stem including multiple system atrophy, Leigh syndrome, and Parkinson disease can be accompanied by CSA. Prevalence rates vary depending on the severity of the disease. In one study, 80% of subjects had sleep apnea, of whom 20% had CSA. In patients with multiple sclerosis, a demyelinating disease that can affect both the brain stem and spinal cord, CSA may develop in those with medullary lesions affecting the respiratory neurons. The prevalence of CSA in such patients is, however, unknown.
Tumors.
There have been fewer than a dozen documented cases of CSA associated with brain stem tumors, suggesting that tumors are a rare cause of hypoventilation with CSA. Tumors such as gliomas, meningiomas, astrocytomas, or neurofibromas have been implicated. The tumor type is probably less important than its location, which usually involves the medulla or pons. In a retrospective 8-year series of children with brain stem tumors referred for assessment to a sleep clinic, CSA was found in 14% (2 out of 14).
Cerebrovascular Disease.
CSA can also result from stroke. The stroke location associated with hypercapnic CSA is usually the nucleus tractus solitarius region of the medulla responsible for neural output to the diaphragm. Prevalence is probably low because there are only a small number of cases reported in the literature. Interestingly, in Fabry disease, an X-linked recessive multisystem disease characterized by vasculopathic changes and ischemia, CSA was reported in 22% of those with white matter lesions in the brain stem.
Neuromuscular Diseases.
Muscular dystrophies, myotonic dystrophy, and other types of inherited and acquired myopathies may result in hypoventilation and hypercapnic CSA. These diseases impair inspiratory muscle function including the diaphragm, leading to alveolar hypoventilation. At the transition from wakefulness to sleep, the associated reduction in ventilatory response to carbon dioxide and hypoxia, combined with the loss of respiratory muscle tone can lead to apneas without respiratory effort (i.e., CSA). Although some central output to the respiratory muscles may be present, and therefore, strictly speaking, events may not be central in origin, the muscles are unable to respond, so there is either markedly reduced or no muscle force generation. The resulting hypopneas and apneas have the appearance of central events. From the clinical viewpoint, it is reasonable to classify these as hypercapnic CSA because their treatment is similar to that for CSA arising from an absence of central drive.
Many of these disorders present in childhood. Although phenotypically variable, most individuals develop progressive muscular weakness and hypoventilation over time. In addition to the high prevalence of hypoventilation, between 20% and 60% of both adults and children develop CSA. When there is associated cardiomyopathy and heart failure, nonhypercapnic Cheynes-Stokes respiration (CSR)–CSA may also be seen in myotonic dystrophy or Duchenne muscular dystrophy.
Diseases of the motor neurons include amyotrophic lateral sclerosis, spinal muscular atrophy, and postpolio syndrome. With degeneration of the respiratory motor neurons, there can be hypoventilation and CSA. The prevalence of CSA is reported to be approximately 18% in amyotrophic lateral sclerosis and approximately 5% in postpolio syndrome.
Myasthenia gravis is a disease of the postsynaptic neuromuscular junction. The reported prevalence of CSA in myasthenia gravis is highly variable (0% to 35%) and is probably dependent on disease severity.
Primary Hypercapnic Central Sleep Apnea
Primary Central Alveolar Hypoventilation Syndrome.
This rare syndrome presents as chronic hypercapnic respiratory failure with normal respiratory mechanics, chest wall configuration, and muscle strength in the absence of any of the previously mentioned secondary causes. The primary abnormality giving rise to hypoventilation is reduced sensitivity of the central and peripheral chemoreceptors. In some cases, this may be due to CCHS. There have only been a few cases of CSA described in association with primary central alveolar hypoventilation syndrome. This diagnosis is made only following the exclusion of other causes of CSA and hypoventilation syndromes, including CCHS.
Clinical Features
As indicated in Table 89-1 , patients with hypercapnic CSA characteristically present with a clinical picture dominated by symptoms and sequelae of chronic respiratory failure with hypercapnia and hypoxemia that can include cyanosis, polycythemia, peripheral edema, and cor pulmonale. Patients may present at variable time points following the onset of the underlying disease. Symptoms suggestive of CSA may include snoring, restless sleep, morning headaches, excessive daytime sleepiness, and fatigue. However, some patients with hypercapnic CSA may not have symptoms suggestive of a sleep apnea syndrome.
Treatment
The initial approach to treatment of hypercapnic CSA is to determine the underlying cause. In some cases, treatment and/or correction of the underlying etiology will alleviate the CSA. For example, in those with tumors compressing the pons or medulla, resection may abolish CSA. Surgical treatment of Chiari II malformations is dependent on the severity of the malformation, and despite posterior fossa decompression for the Chiari II malformation, CSA will often persist. In acute stroke patients, no specific intervention is usually required for CSA because recovery may accompany improvement from the stroke. However, if respiratory failure and CSA persist, specific therapy for these conditions may be indicated as described later. For those with neuromuscular disease, pharmacologic therapies available for some inherited myopathies or myasthenia gravis may also alleviate CSA by improving muscle strength.
In most patients the management of CSA is the same as for chronic alveolar hypoventilation syndromes. However, few studies have specifically addressed the treatment of hypercapnic CSA. The main aim of treatment is to augment ventilation and thereby improve gas exchange and abolish CSA.
It is important that patients be cautioned against the use of sedative medications that can induce acute respiratory failure and aggravate CSA. In patients with CSA in association with central alveolar hypoventilation, a trial of the respiratory stimulant, medroxyprogesterone, can be tried but is usually successful in only a minority of patients. Supplemental nocturnal oxygen may alleviate hypoxia and CSA and may even lower P co 2 if hypoxia is a cause of central nervous system depression. However, supplemental oxygen can also abolish hypoxic respiratory drive and worsen CSA. Therefore, a trial of supplemental oxygen should be accompanied by careful monitoring of P co 2 and either P o 2 or arterial S o 2 .
Where pharmacologic therapies are unavailable or ineffective, noninvasive ventilation is often indicated, either via bilevel positive airway pressure support ventilation (BiPAP) or volume-cycled ventilators. The aim of noninvasive ventilation is to reduce P co 2 below 45 mm Hg while awake and below 50 mm Hg while asleep while maintaining arterial S o 2 greater than or equal to 90%. This is best achieved by performing polysomnography during a trial of noninvasive ventilation in which transcutaneous P co 2 or fraction of end-tidal CO 2 and arterial S o 2 are monitored. It is sometimes possible to maintain normal gas exchange during both sleep and wakefulness through noninvasive ventilation only during sleep. If the underlying disease progresses despite the previously mentioned measures, tracheotomy and invasive mechanical ventilation may be required. Unfortunately, there are no randomized trials or long-term observational studies that have determined the effects of noninvasive and invasive mechanical ventilation on morbidity, mortality, and quality of life in patients with hypercapnic CSA.
Nonhypercapnic Central Sleep Apnea
The clinical picture of nonhypercapnic CSA differs in many ways from that of hypercapnic CSA (see Table 89-1 ). This table is based mainly on symptoms related to idiopathic CSA. Nevertheless, symptoms related to the many secondary forms of nonhypercapnic CSA are, in general, similar.
Secondary Forms of Nonhypercapnic Central Sleep Apnea
Central Sleep Apnea in Association with Heart Failure—Cheyne-Stokes Respiration.
CSA in heart failure is manifest by a Cheyne-Stokes respiratory pattern that can exist during both sleep and wakefulness. Because this chapter focuses on central apnea during sleep, we discuss Cheyne-Stokes respiration with central apneas during sleep and refer to it as CSR-CSA (see also Chapter 85 ). CSR-CSA is distinguished from other forms of CSA and periodic breathing by its characteristic crescendo-decrescendo pattern of tidal volume in which hyperpneas are markedly prolonged compared with those without heart failure owing to reduced cardiac output and prolonged lung to chemoreceptor circulation time (upper panel of Fig. 89-4 ). Therefore, a finding of CSR-CSA on a polysomnogram is highly suggestive of low cardiac output and the presence of cardiac dysfunction.

Mechanisms that mediate CSR-CSA remain incompletely understood. However, as in all other forms of nonhypercapnic CSA, central to the process is respiratory control instability resulting from the dependence of the sleep state on metabolic control of ventilation, especially P co 2 . A proposed pathophysiologic scheme for CSR-CSA is illustrated in Figure 89-5 .

In heart failure, a key factor destabilizing the respiratory control system and predisposing to CSR-CSA is the presence of chronic hyperventilation that lowers arterial P co 2 both during wakefulness and sleep and maintains it close to the apnea threshold. Because arterial P co 2 is closer to the apnea threshold than normal, small perturbations, such as arousals from sleep that augment ventilation, may be sufficient to drive arterial P co 2 below the apnea threshold and trigger central apneas. The main factor contributing to this instability and hyperventilation is increased loop gain. Loop gain is an engineering term first used in the context of sleep-disordered breathing in a mathematical model developed to explain factors involved in the instability within the respiratory control system in patients with CSR-CSA. Simply put, it is the ratio of ventilatory output to a given stimulus. Ventilation becomes unstable when loop gain is elevated so that the ventilatory output for a given stimulus is higher than normal. So, for example, if central sensitivity to carbon dioxide is augmented, any given increase in P co 2 will cause a greater than normal increase in ventilation that can then drive P co 2 close to, or below, the apnea threshold.
Although a detailed discussion of loop gain is beyond the scope of this chapter, several factors interact to increase the tendency to respiratory instability and hyperventilation: (1) increased peripheral and central chemoresponsiveness ; (2) elevated pulmonary capillary wedge pressure that causes pulmonary congestion and activation of the pulmonary juxtacapillary irritant receptors that augment central drive and ventilation ; (3) impaired cerebral vascular reactivity and blood flow responses to changes in the arterial P co 2 , leading to instability of breathing. Whereas this has been proposed as a mechanism for ventilatory instability, it requires a differential effect of P co 2 on the cerebral arterial and venous vessels that has not been fully demonstrated or explained. Consequently, this potential mechanism remains controversial and not fully established; (4) sleep state instability in which arousals from sleep during the hyperpneic phase of CSR-CSA cause a transient awakening with an associated engagement of the wakefulness drive to breathe, increased chemosensitivity, and a lower arterial P co 2 apnea threshold. Unlike in OSA, in which arousals happen at apnea termination and contribute to airway opening and resumption of airflow, arousals following central apnea generally develop several seconds after resumption of airflow but before the peak of hyperventilation. Thus they appear not to have the same protective role as they do in OSA. Indeed, recurrent arousals during the ventilatory phase have been shown to trigger and propagate CSR-CSA.
CSR-CSA is typically seen during non-REM sleep and resolves or becomes less prominent in REM sleep because of several reasons. First, breathing is predominantly under metabolic control during non-REM sleep and is therefore highly dependent on arterial P co 2 . Thus, reductions in arterial P co 2 below the apnea threshold will invariably give rise to central apneas. However, breathing is largely independent of metabolic control during REM sleep, so it is not nearly as dependent on arterial P co 2 as in non-REM sleep. Second, ventilatory responses to carbon dioxide and hypoxia are brisker in non-REM than in REM sleep, so the potential to hyperventilate is greater in the former than in the latter state. Third, arousal thresholds for arterial P co 2 and arterial P o 2 are significantly lower in non-REM than in REM sleep. Thus, people are more arousable and therefore more prone to respiratory control system instability in non-REM than in REM sleep. Fourth, the respiratory muscles, save for the diaphragm, are essentially paralyzed in REM but not non-REM sleep. Thus, for any given chemical (e.g., arterial P co 2 ) or nonchemical (e.g., arousal) stimulus, the augmentation in ventilation will be greater in non-REM than in REM sleep, making hyperventilation more likely in the former state.
It appears that low cardiac output and prolonged circulation time are not crucial to the pathogenesis of the central apneas because there is evidence that cardiac output in heart failure patients with CSR-CSA does not differ significantly from that in heart failure patients without CSR-CSA. Moreover, in dogs, prolongation of lung-to-carotid body circulation time only precipitated period breathing in a minority of experiments and then only when circulation times approached 1 minute or longer, a time far exceeding that observed in human heart failure. However, these factors play a role in sculpting the crescendo-decrescendo pattern of hyperpnea because the rate of rise of tidal volume and the duration of the hyperpnea are directly proportional to lung to chemoreceptor circulation time and indirectly proportional to cardiac output. As a consequence, in patients with heart failure, cardiac output is lower and lung-to-chemoreceptor circulation time, hyperpnea, and periodic breathing cycle duration of CSR-CSA are longer than they are in CSA in patients without heart failure (see Fig. 89-4 ).
Heart failure is a prevalent condition affecting approximately 1.5% of the general population. Among patients with symptomatic heart failure, earlier studies involving only men reported a prevalence of CSR-CSA of approximately 40%. However, more recent epidemiologic studies involving both men and women with heart failure have shown a substantially lower prevalence of CSR-CSA of 15% to 26%, probably because CSR-CSA is uncommon in women. CSR-CSA also appears to be common in patients with asymptomatic left ventricular systolic dysfunction. Risk factors for CSR-CSA include older age (>60), male sex, atrial fibrillation, awake hypocapnia (P co 2 ≤ 38 mm Hg), diuretic use, more severe heart failure defined by lower left ventricular ejection fraction, higher New York Heart Association class, higher brain natriuretic peptide level, higher pulmonary capillary wedge pressure, and higher left ventricular end-diastolic volume.
OSA and CSA can coexist in patients with heart failure. In a significant minority of patients, there may be a shift between the two types of apnea overnight or over longer periods. The shift from predominantly obstructive to central events is associated with reductions in the P co 2 and prolongation of circulation time and the hyperpnea phase. This suggests a deterioration in cardiac output and elevation in left ventricular filling pressure as a result of the adverse hemodynamic effects of OSA. Conversely, the shift from central to OSA is associated with improvements in left ventricular ejection fraction and shortening of circulation time. These observations suggest a pathophysiologic interaction between heart failure and sleep apnea in that sleep apnea can influence cardiac function and heart function can influence sleep apnea type. Thus, in some heart failure patients, OSA and CSA may be part of a spectrum of sleep-related breathing disorders that can shift according to underlying cardiovascular function.
One factor that may contribute to the pathogenesis of both OSA and CSR-CSA, as well as to shifts in predominant apnea type over time in patients with heart failure, is fluid retention in the legs during the day and its overnight rostral redistribution during the night. Strong relationships between the amount of fluid displaced from the legs overnight and the AHI have been described in heart failure patients with either OSA or CSA. In the case of OSA, with the fluid shift, there was an increase in neck circumference that correlated strongly with the AHI. These observations suggest that fluid accumulation in the neck was contributing to severity of OSA likely by increasing peripharyngeal tissue pressure, thereby increasing its collapsibility. In the case of CSA, with the fluid shift, there was a decrease in nocturnal P co 2 , suggesting that some of the fluid was redistributed into the lungs, where it stimulated pulmonary irritant receptors and thereby caused hyperventilation and reductions in the arterial P co 2 close to or below the apneic threshold. In addition, the magnitude of the fluid shift from the legs was greater in those with CSA than in those with OSA. Thus, it is possible that varying degrees of rostral fluid shift could lead to changes in predominant apnea type over time. The degree of nocturnal rostral fluid shift is related directly to time spent sitting in the day and inversely to physical fitness.
Clinical Features
Although orthopnea, paroxysmal nocturnal dyspnea, witnessed apnea, fatigue, and insomnia are common in those with CSR-CSA, no specific constellation of symptoms has been identified in association with CSR-CSA. For example, patients with CSR-CSA do not usually have snoring or excessive daytime sleepiness. Nor is there clear evidence that CSR-CSA has an adverse impact on quality of life.
It is still a matter of debate as to whether CSR-CSA is merely a manifestation of elevated left ventricular filling pressures and poor cardiac function or whether it has an adverse effect on cardiac function and prognosis independently of other risk factors. The balance of the evidence suggests an adverse effect of CSR-CSA on prognosis. Most, but not all, single-center observational studies have reported a twofold to threefold increased risk of death or need for cardiac transplantation in association with CSR-CSA. Caution is recommended in interpreting these studies because patient populations were generally small and definitions and quantification of CSR-CSA were inconsistent. Because several of these studies were reported before widespread treatment of heart failure with β-blockers, implantable cardiac defibrillators, and cardiac resynchronization therapy, their findings may no longer be applicable.
One factor that might contribute to poor prognosis in CSR-CSA is elevated sympathetic nervous system activity that is known to have a deleterious effect on the prognosis of patients with heart failure. There is evidence that CSR-CSA increases sympathetic nervous activity both during sleep and during wakefulness, probably via intermittent apnea-related hypoxia and arousals from sleep. High sympathetic nervous activity induces activation of the aldosterone-renin pathway and reduces myocardial contractility over time due to abnormalities in calcium handling with precipitation of cardiac arrhythmias. It is this combination of autonomic dysfunction and propensity to arrhythmias and sudden death that are the presumed mechanisms by which CSA contributes to increased mortality in heart failure.
On the other hand, there is some evidence that CSR-CSA does not cause adverse hemodynamic effects in patients with heart failure. A recent study demonstrated that in contrast to obstructive apneas, during which stroke volume and cardiac output decrease, during central apneas, stroke volume and cardiac output actually increase slightly. The cause of this effect was not identified, but this observation raises the possibility that under certain circumstances, CSR-CSA may provide some compensatory effect for low cardiac output and may not be entirely detrimental to cardiovascular function.
Treatment
Because few symptoms have been attributed to CSR-CSA, symptomatic targets for therapy are difficult to identify. However, because CSR-CSA is associated with increased risk of morbidity and mortality, a logical aim in treating CSR-CSA would be to reduce morbidity or mortality. Nevertheless, no treatments have been shown to affect these outcomes in randomized trials. Thus, because there are no clear-cut indications for treating CSR-CSA, a decision to treat CSR-CSA rests on clinical judgment and the following possibilities can be considered with the proviso that therapies that reduce the severity of CSR-CSA may not lead to improvements in patient symptoms, quality of life, or long-term outcomes. All interventions in current use for CSR-CSA require further study to assess their long-term effectiveness and safety.
Treatment of Heart Failure
Because CSR-CSA arises as a consequence of heart failure, it is reasonable to optimize pharmacologic and device treatments for the underlying heart failure. A few nonrandomized observational studies suggest that angiotensin-converting enzyme inhibitors, β-blockers, and cardiac resynchronization therapy may reduce the severity of CSR-CSA in CHF. However, these findings have not been confirmed in randomized trials, and one large epidemiologic study showed no reduction in frequency or severity of CSR-CSA with institution of β-blockers. In one study, atrial overdrive pacing led to a reduction in the AHI in patients with bradyarrhythmias and CSA, but without heart failure. However, these findings have not been reproduced. In another small study, it was reported that cardiac transplantation for end-stage heart failure reduced severity of CSR-CSA. Although consideration can be given to the implementation of one or more of the previously mentioned approaches, none of them is specifically indicated for therapy of CSR-CSA, and their use should be reserved to treat the underlying heart failure as required.
Positive Airway Pressure
Continuous Positive Airway Pressure.
Continuous positive airway pressure (CPAP) has been studied extensively in patients with heart failure and CSR-CSA. Its acute application in patients with heart failure increases intrathoracic pressure, thereby reducing right and left ventricular volumes (i.e., preload), left ventricular transmural pressure (i.e., afterload), and the work of breathing by unloading the inspiratory muscles. It also augments stroke volume and cardiac output in those with elevated left ventricular filling pressures but has the opposite effect in those with normal or reduced left ventricular filling pressures.
Several small randomized trials in heart failure patients with CSR-CSA lasting 1 to 3 months have demonstrated that CPAP reduces the AHI by 50% to 60%. The means by which it reduces the AHI are not well understood but may include raising lung volume, thereby increasing the lung O 2 reservoir and thus dampening fluctuations in Pa o 2 , reducing lung water and thus pulmonary irritant receptor stimulation, and reducing ventilation and allowing arterial P co 2 to rise above the apnea threshold. Other small randomized trials in heart failure patients with CSR-CSA also demonstrated that CPAP increases left ventricular ejection fraction, reduces mitral regurgitation, and decreases sympathetic nervous system activity.
The largest randomized trial involving CPAP was the Canadian Positive Airway Pressure for Patients with Central Sleep Apnea and Heart Failure (CANPAP), which involved 258 patients over a mean and maximum follow-up of 24 and 64 months. CPAP reduced the AHI by approximately 55%, improved left ventricular ejection fraction and 6-minute walking distance, and reduced sympathetic nervous system activity. However, it had no effect on the primary outcome, which was heart-transplant free survival. Therefore, it cannot be recommended for routine use as therapy for CSR-CSA. However, in a post hoc analysis of the trial, patients in whom CPAP reduced the AHI to less than 15 had significantly higher heart-transplant free survival than the control group and the CPAP subgroup in whom the AHI remained above 15. These results suggest that a trial of CPAP may be given to heart failure patients with CSR-CSA and, if their AHI is reduced to below 15, then CPAP can be continued because of its potential to reduce morbidity and mortality. However, if the AHI remains above 15, CPAP should be stopped because of the potential for harm.
Adaptive Servoventilation.
Adaptive servoventilation (ASV) is a form of positive airway pressure specifically designed to alleviate CSR-CSA. For CSR-CSA, ASV is analogous to a cardiac pacemaker: When it detects a central apnea, it provides intermittent pressure support ventilation, but when it detects that the patient is breathing spontaneously, inspiratory assist is turned off. In heart failure patients with CSR-CSA, it causes a greater fall in the AHI than CPAP, bilevel positive airway pressure, and supplemental O 2. However, the clinical effects of ASV in heart failure patients with CSR-CSA are inconsistent. In one randomized trial involving 72 patients over 3 months, ASV did not cause any significant changes in left ventricular ejection fraction, NT-proBNP level, or quality of life compared with control. In another randomized trial involving 26 patients over 4 weeks, ASV improved an objective measure of alertness and reduced BNP levels but had no effect on LVEF, subjective daytime sleepiness, simulated driving performance, or quality of life. There have been no long-term randomized trials of ASV that have assessed effects on morbidity or mortality. Accordingly, there are insufficient clinical outcomes data from randomized trials to support the use of ASV to treat CSR-CSA in patients with heart failure. Further evidence from large-scale randomized trials is required.
Supplemental Oxygen.
Supplemental oxygen reduces central apnea–related dips in arterial P o 2 , thereby reducing peripheral chemoreceptor stimulation; this reduces loop gain and lessens the chance of ventilatory overshoot and dips in arterial P co 2 below the apnea threshold. Where P co 2 has been measured during supplemental oxygen administration, it was reported to increase when the AHI was markedly reduced, but remain the same when the AHI only decreased marginally, suggesting that one of the mechanisms by which oxygen attenuates CSR-CSA is via dampening of ventilation and raising P co 2 above the apnea threshold.
Small randomized trials, from one night to 1 month duration, have demonstrated that nocturnal oxygen reduces AHI by approximately 50% in heart failure patients with CSR-CSA. However, the effects of oxygen on physiologic and clinical outcomes have been inconsistent. Staniforth and colleagues found that supplemental oxygen for 1 month reduced overnight urinary norepinephrine excretion but had no effect on daytime plasma norepinephrine, brain natriuretic peptide, neurocognitive function, sleepiness, or quality of life. In another randomized trial, Andreas and colleagues reported that administration of nocturnal oxygen for 7 days to 22 patients with heart failure improved peak oxygen consumption and ventilatory efficiency but had no effect on quality of life. Arzt and colleagues allocated 10 consecutive patients to nocturnal oxygen and the next 16 consecutive patients to CPAP at 8 to 10 cm H 2 O for 3 months. Both CPAP and oxygen reduced the AHI by 67%, but only CPAP improved ventilatory efficiency and LVEF. Neither intervention had any effect on peak exercise oxygen consumption. The Home Oxygen Trial (HOT) randomized 51 patients to receive or not receive supplemental oxygen over 1 year. Those who received oxygen had a significant reduction in severity of CSR-CSA and a significant improvement in quality of life as measured by the Specific Activity Scale compared with the control group. However, there was no difference in cardiac event rates among the groups.
Although oxygen attenuates CSR-CSA in patients with heart failure and can reduce nocturnal sympathetic activity, there is no consistent evidence that it improves cardiovascular function or clinical outcomes in such patients. Moreover, administration of supplemental oxygen to patients with heart failure could cause hyperoxia, and by doing so, induce oxidative stress that can exert adverse hemodynamic effects, such as raising vascular resistance, blood pressure, and left ventricular filling pressure, and lowering cardiac output. Therefore, there is currently insufficient evidence to support the use of oxygen to treat CSR-CSA in patients with heart failure.
Respiratory Stimulants.
Acetazolamide is a carbonic anhydrase inhibitor that causes a metabolic acidosis that increases the stimulus to breathe while lowering the arterial P co 2 apneic threshold. One small 6-day randomized trial demonstrated a modest (38%) reduction in the AHI in heart failure patients with CSR-CSA in association with reductions in daytime sleepiness and fatigue. Theophylline is an adenosine antagonist that stimulates central respiratory drive and augments cardiac contractility. In a 5-day randomized trial involving 15 patients with heart failure and CSR-CSA, theophylline reduced the AHI but did not improve left ventricular ejection fraction. However, although once used widely for therapy of acute heart failure, theophylline is no longer used for this purpose because it increases the risk of cardiac arrhythmias and sudden death. Consequently, neither of these agents can be recommended for therapy of CSR-CSA.
Carbon Dioxide.
Raising arterial P co 2 above the apnea threshold either by inhalation of carbon dioxide or the addition of dead space abolishes CSR-CSA instantaneously in patients with heart failure ( Fig. 89-6 ). However, there is no evidence that raising P co 2 through these means provides any clinical benefits. Moreover, because carbon dioxide increases sympathetic nervous activity and disrupts sleep and because no long-term studies have demonstrated its safety or clinical efficacy, it cannot be recommended for therapy of CSR-CSA.
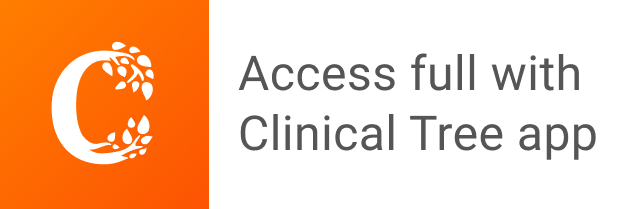