Fig. 12.1
A typical mammalian cell. The intracellular environment is separated from the extracellular environment by a lipid bilayer membrane (see area encircled in figure). Each cell contains a nucleus containing chromosomes and a collection of organelles to support biosynthetic and other “housekeeping” tasks. Shown here are the endoplasmic reticulum, ribosomes, mitochondria, Golgi apparatus, lysosomes, various ion channels, and biochemical receptors
12.2 Cardiac Muscle Cell Morphology
Muscle cells are similar in that they contain these common organelles but distinct in that they also include an elaborate protein scaffold within the cell that is anchored to the cell membrane and the extracellular matrix of connective tissue (Fig. 12.2). Force generation arising from protein–protein interactions within the internal protein lattice leads to the contraction of the cells and pumping of blood by the heart. Mammalian cardiac cells are roughly cylindrical but may also include short branch-like projections. The cells have an asymmetric profile with diameters in the range of 10–20 μm and lengths on the order of 50–100 μm. Force is produced primarily along the long axis of the cell. Most of the internal volume of myocytes is devoted to a cytoskeletal lattice of contractile proteins whose liquid crystalline order gives rise to a striated appearance under the microscope (Figs. 12.2 and 12.3). As with other cell types, the membrane bilayer contains a collection of ion channels and ion pumps and receptor proteins. In addition, the membranes of cardiac muscle cells contain some proteins which connect cardiac myocytes to one another as mechanical partners and other proteins which facilitate cell-to-cell electrical communications .
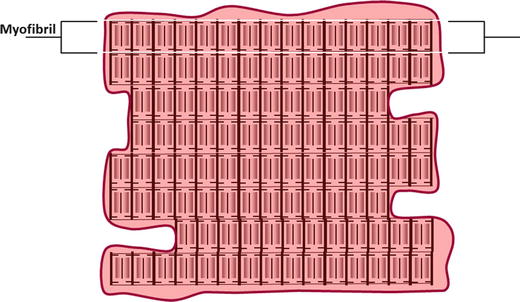
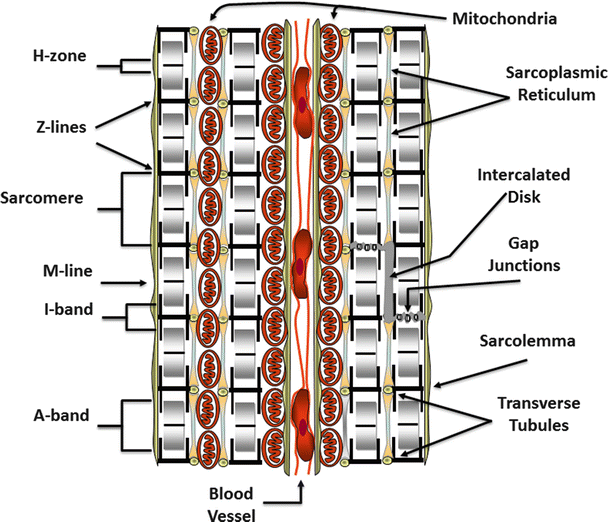
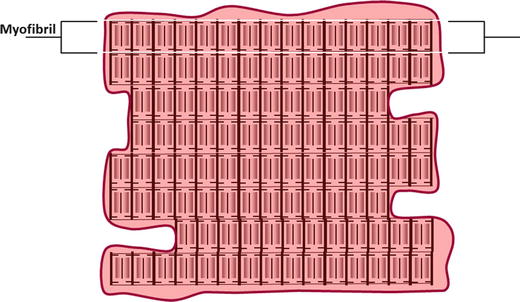
Fig. 12.2
A typical cardiac cell. The intracellular space is largely filled with contractile structures called myofibrils. The nucleus and other organelles that support cellular function are present but are often crowded to the periphery by the contractile apparatus
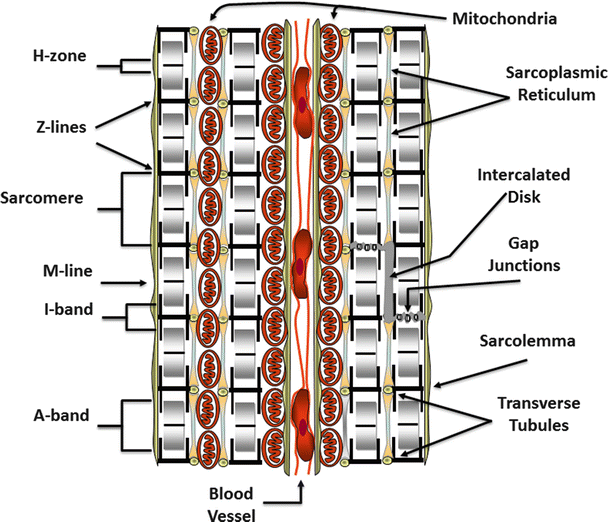
Fig. 12.3
A cross section of cardiac tissue showing two cells separated by a blood vessel containing red blood cells. The repeating sarcomeric structure of the myofibrils and the names of the sarcomeric landmarks are highlighted on the left of the figure. On the right of the figure, the legend points out the membrane specializations of the cell. These include the intercalated disks, gap junctions, the transverse tubules that punctuate the sarcolemma (plasma membrane), and the sarcoplasmic reticulum. Also shown are mitochondria compacted into a limited space because of the abundance of the myofibrils
12.3 Cardiac Cell Membranes
The surface (or plasma) membranes of cardiac cells are punctuated by openings of membrane-lined channels, the transverse tubules (or T-tubules), that pass through the cell and are filled with extracellular fluid (Fig. 12.4). As they traverse the cell, individual T-tubule passages will branch and connect to other transverse tubule channels forming a reticular network that encircles the internal contractile structures known as myofibrils. Abutting the T-tubules, in the sarcoplasm (cytoplasm) of the myocytes, is the sarcoplasmic (endoplasmic) reticulum, a cytosolic organelle that is specialized to store calcium. The T-tubules provide a pathway for conduction of action potentials through the entire thickness of a cardiac myocyte. Their close association with the sarcoplasmic reticulum helps to couple cardiac action potentials to the release of calcium from internal stores. This arrangement of the T-tubules with the sarcoplasmic reticulum is also found in skeletal muscle, but not within smooth muscles .
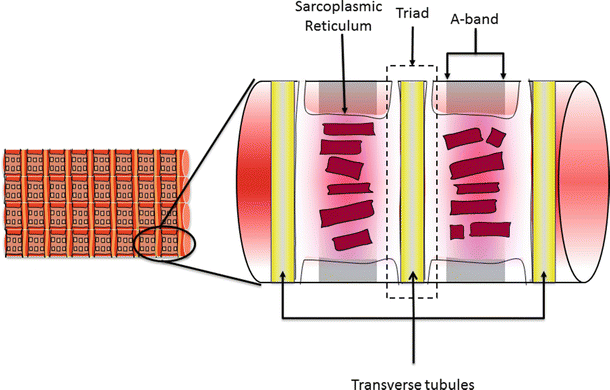
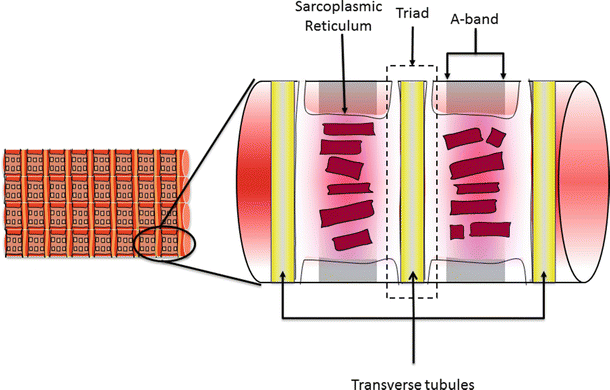
Fig. 12.4
A section of a cardiac cell showing the relationship of the sarcoplasmic reticulum and the transverse tubules (T-tubules). The sarcoplasmic reticulum surrounds each myofibril and lays adjacent to the T-tubules as they pass through a cardiac cell. The junction of the T-tubule with the adjacent sarcoplasmic reticulum is referred to as the triad. This close proximity allows the action potentials that pass through the T-tubular system to influence calcium release from the sarcoplasmic reticulum
12.4 Intercalated Disks
A structure referred to as the intercalated disk forms strong mechanical links between myocytes (Fig. 12.5). The intercalated disk structures are formed by the association of membrane-bound proteins projecting from the surfaces of the neighboring cardiomyocytes. The protein components of these membrane plaques include: N-cadherin, desmin, vinculin, α- and β-catenin, desmoplakin-1, desmocollin-2, and plakoglobin-2 [1, 2]. The tight cell-to-cell coupling of the intercalated disks contributes structural integrity to branches of myocardial cells. This connection of the cardiac myocytes facilitates some lateral shifting and the interdigitation of the cells. However, longitudinal shifting of cardiac myocytes relative to one another is practically impossible (Fig. 12.5). Importantly, it is the structural integrity of the intercalated disks between individual cells that allows force to be transmitted across the myocardium .
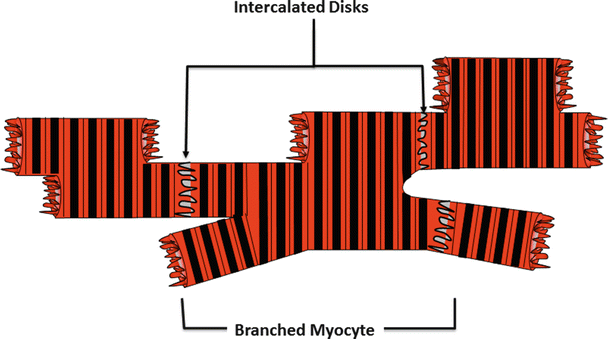
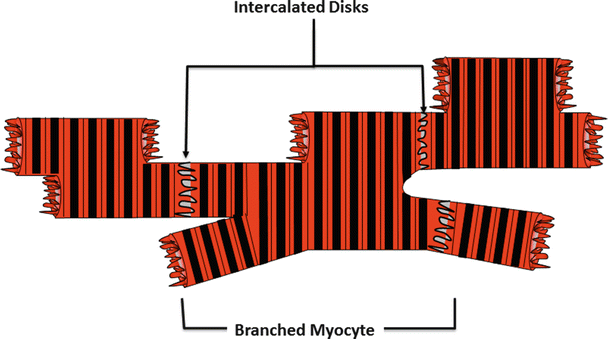
Fig. 12.5
A collection of interconnected cardiac muscle cells showing their characteristic branched structure. At the interface of adjoining cells, there is an interconnection of membrane-bound proteins known as the intercalated disk. This structure mechanically couples the cardiac cells so that the forces generated by each cell are communicated through the vessels of the heart
12.5 Gap Junctions
Gap junctions form electrical connections between cardiac cells [3]. Membrane proteins known as connexins form six-membered rings called connexons imbedded in the sarcolemma of cardiac cells (Fig. 12.6a). The connexons on the surface of one cell dock with connexons on the surface of a neighboring cell. A conformational change within the proteins of docked connexons results in openings of aqueous pores or gap junctions (Fig. 12.6b). When gap junctions are open, they provide for direct electrical and small molecule communication between the sarcoplasmic spaces of adjoining cells, creating a functional syncytium or network of synchronized cells. This connectivity allows activation signals to be passed from cell to cell in cardiac tissue. Electrical depolarizations can then pass from cell to cell through these gap junctions; this facilitates the seemingly simultaneous, coordinated contractions of cardiac muscle. For more details on movement of electrical signals through heart tissue, the reader is referred to Chap. 13 .
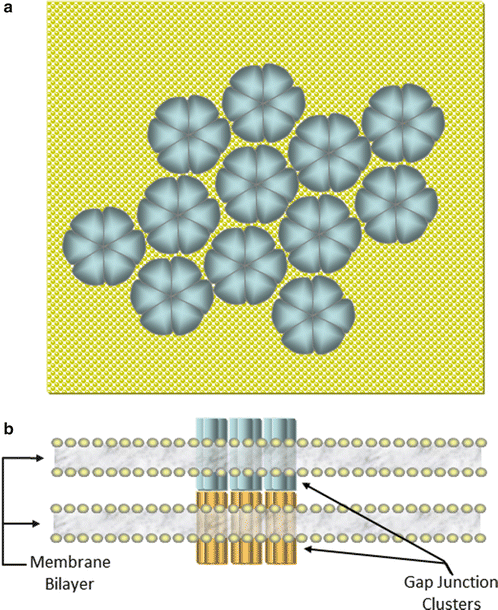
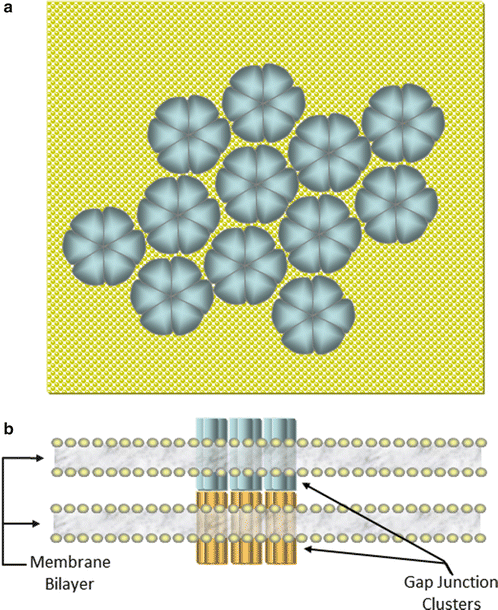
Fig. 12.6
(a) Overhead representation of a plaque of connexons on a cardiac membrane . Six connexins form each pore structure (connexons) on the membrane surface which cluster in the intercalated disk regions at cell–cell interfaces. (b) Side view of the interface between two cells showing the docking of connexons to form a gap junction between adjacent cells
12.6 Myofibrillar Structure
The arrangement of contractile proteins in cardiac muscle cells is similar to that found in skeletal muscle. Many contractile protein assemblies, known as myofibrils, run parallel to one another along the long axes of the myocardial cells (Fig. 12.2). Myofibrils fill most of the cytoplasmic space of each cardiac myocyte, with the remainder of these cells occupied by the normal intracellular machinery (Fig. 12.3). Each myofibril is composed of a serial array of contractile elements called sarcomeres, defined as the smallest functional units within muscle (Figs. 12.3 and 12.7). It is the arrangement of contractile proteins into the sarcomeres that gives cardiac and skeletal muscle the characteristic striated appearance under microscopic examination. At regular intervals along each myofibril, a transverse matrix composed primarily of the proteins α-actinin and actin forms boundaries known as Z–disks (or Z-lines; Fig. 12.7). A sarcomere is defined as the arrangement of contractile proteins that resides between two consecutive Z-disks along a myofibril. Actin filaments anchored on each face of a Z-disk extend for 1 μm toward the center of adjacent sarcomeres (thin filaments). Thick filaments of the protein myosin sit in the center of each sarcomere and extend toward the Z-disks at the ends of the sarcomere (thick filament length ~1.6 μm). The thick filaments are connected at their centers by a protein matrix referred to as the M–line (or M-disk) and tethered to the Z-disks by filaments of the protein titin. The region of the sarcomere in which the myosin filaments reside is known as the A–band (Figs. 12.3 and 12.7). The area between A-bands is known as the I–band; each I-band is bisected by a Z-line and is traversed by the actin thin filaments (Figs. 12.3 and 12.7) .
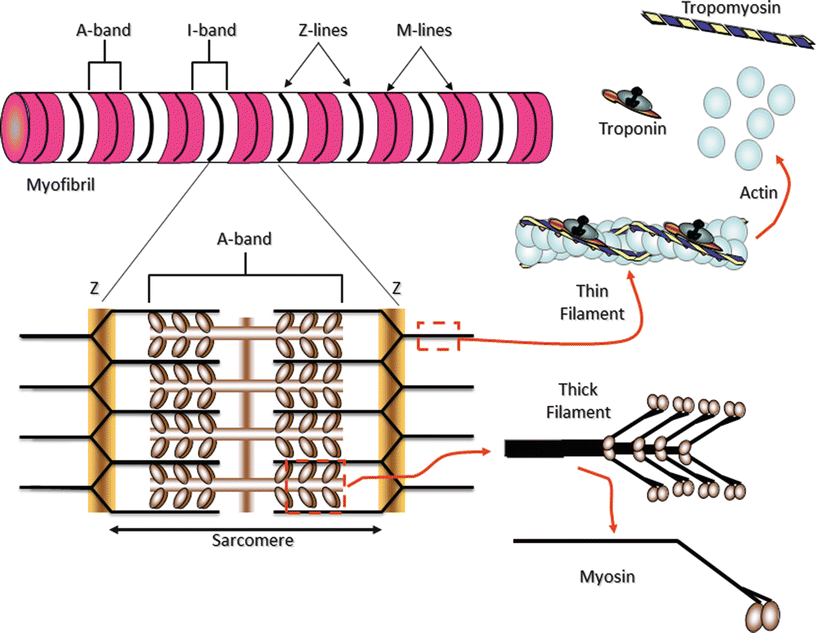
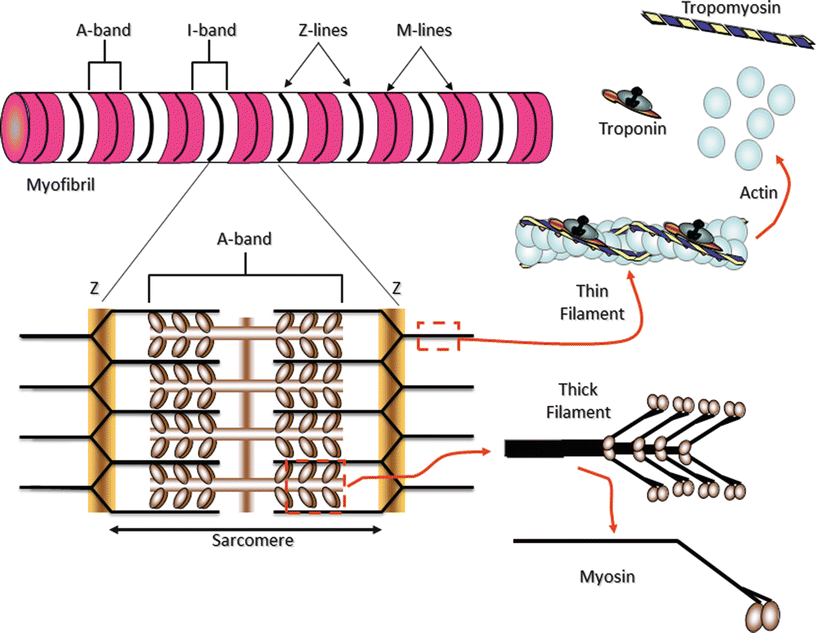
Fig. 12.7
Myofibrils are constructed of repeating sarcomeres within cardiac muscle cells. Each sarcomere is defined as the structures bounded on each end by Z-disks (Z-lines). Thin filaments of the protein actin are attached to the Z-line and reach toward the center of each sarcomere. Two regulatory proteins are found on the double-stranded actin thin filaments—tropomyosin and troponin. Tropomyosin is a double-stranded a-helical protein dimer that binds across seven actin monomers on the thin filament obscuring a binding site for myosin. Troponin is a three subunit globular protein that binds one per tropomyosin. Thick filaments of the protein myosin are found in the center of the sarcomere. The area that contains the thick filaments is also known as the A-band. The myosin molecules are asymmetrically shaped with a coiled-coil “tail” and two globular “head” domains. The head domains bind to actin to form crossbridges between the filaments and generate contractile force
12.7 Thin Filament
As noted above, the principle structural component of the thin filaments is a double-stranded filament of the globular protein actin (Fig. 12.7). The thin filaments also incorporate the regulatory proteins tropomyosin (Tm) and troponin (Tn). Tropomyosin is a double-stranded α-helical coiled-coil protein that spans seven actin monomers (~35 nm). Troponin is a globular protein complex with three subunits: TnC, a calcium-binding subunit; TnI, a subunit which facilitates inhibition of muscle contraction; and TnT, a subunit that connects the troponin complex to tropomyosin and actin. Tropomyosin molecules are aligned end to end around the helical coil of the thin filament with one Tn complex attached to each Tm molecule. In relaxed muscle, the track traced by tropomyosin as it binds to actin on the thin filament impedes the binding of the myosin crossbridge domains (see below) to actin-binding sites [4]. However, upon myocyte activation and the subsequent increase in myoplasmic calcium concentrations, free calcium binds to TnC inducing a conformational change of the entire troponin complex that is transmitted to tropomyosin. Tm then shifts its position on the actin thin filament, revealing the site on actin required for strong myosin binding. Myosin can then bind to the thin filament in a manner conducive to force production. This association of a tropomyosin-troponin complex over seven actin monomers represents a de facto regulatory subunit along the thin filament. The overlap of Tm molecules creates a mechanism for the communication of the activation signal along the thin filament, making the initiation of force generation via this mechanism highly cooperative [4].
This thin filament-based mechanism for the regulation of contraction is also used for the control of skeletal muscle. In contrast, in smooth muscle (e.g., the muscles of the vascular system, gut, and airways), while the regulation of contraction is also calcium dependent, the regulatory protein troponin is absent. The rise in calcium concentration is sensed by the cytosolic protein calmodulin, and activation occurs via a different thick filament-based mechanism.
12.8 Thick Filament
Myosin is the molecular motor protein responsible for force production and movement of muscle cells. The functional protein is a hexamer (six-sub unit) composed of two protein heavy chains and four light chains. Each heavy chain is asymmetrically shaped with a long alpha-helical “tail” and two globular “head” domains (Fig. 12.7). Associated with each globular head domain are two smaller protein components, the “light chains” of myosin. The heavy chain tails form an alpha-helical coil which completes the assembly of the molecule. The coiled tails of myosin self-associate in an anti-parallel manner to form the backbone of the thick filament (Fig. 12.7). This results in a bipolar structure that has a bare zone in the center and the globular “head” domains of the myosin molecules projecting from each end. These head domains contain an actin-binding site and an ATP hydrolysis site. As will be discussed later, the cyclic interaction of these crossbridge forming head domains to the actin thin filaments forming crossbridges provides the underlying mechanism for myocyte contraction .
12.9 Energy Metabolism
ATP production in cardiac muscle is primarily accomplished via oxidative phosphorylation. Oxidative phosphorylation is a multistep enzymatic process that extracts energy from glucose, fatty acids, and other energy-rich compounds and converts it to ATP. The energy extraction and ATP production occur in the mitochondria found in the cytoplasmic space of cardiac myocytes. Intracellular concentrations of ATP hover in the 4–5 millimolar (mM) range with additional energy stored in creatine phosphate which acts as a backup to the ATP supply. Creatine phosphate (~20 mM) can be used to regenerate ATP in a one-step enzymatic process catalyzed by the enzyme creatine kinase. There is an absolute requirement for oxygen in the ATP production mechanism, and it is the reason that blood flow to the myocardium is so critical. The amount of ATP and creatine phosphate normally present is insufficient to power the contractile activities and other uses of ATP in heart cells for more than a few beats. Therefore, continuous delivery of nutrients and oxygen and removal of waste products are crucial processes for the normal functioning of the myocardium. For a more detailed description, see Chap . 21.
< div class='tao-gold-member'>
Only gold members can continue reading. Log In or Register a > to continue
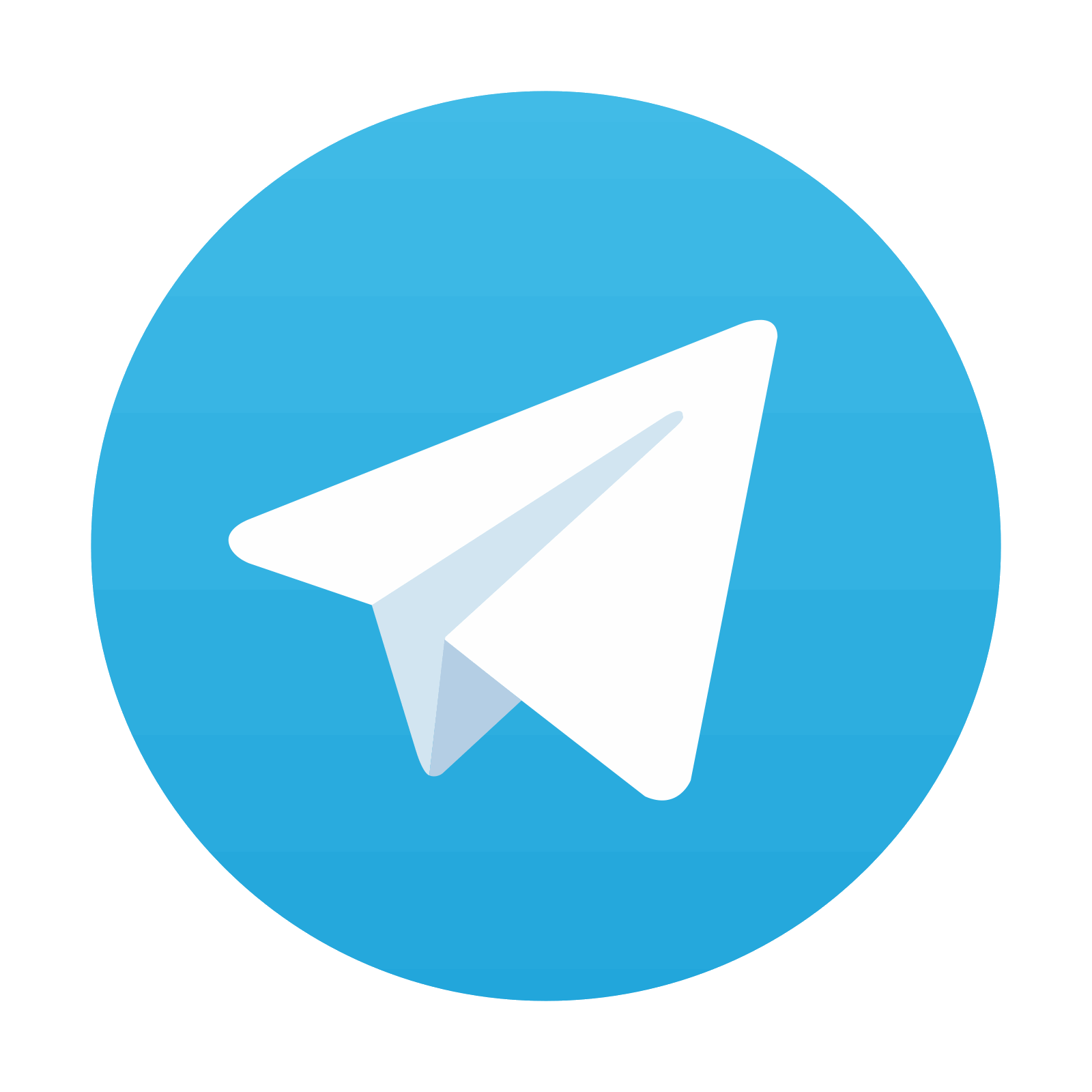
Stay updated, free articles. Join our Telegram channel
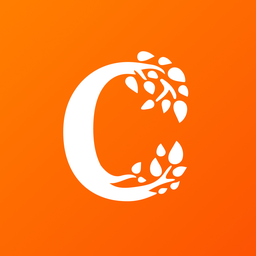
Full access? Get Clinical Tree
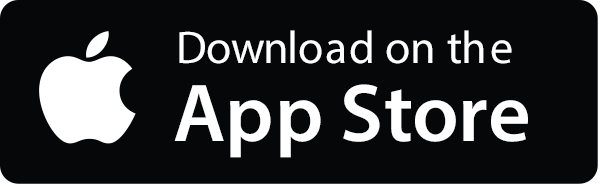
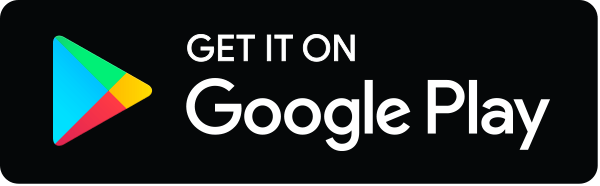