120 Interventional cardiac electrophysiology relies on the ability to deliver energy to myocardial tissue to render it nonexcitable and prevent the generation or propagation of arrhythmias. The goal of catheter-based ablation technologies is to create myocardial lesions that are precise, effective, and permanent, in a reasonable time frame and without collateral damage. This chapter highlights the biophysical principles of lesion formation, reviews currently available catheter ablation technologies, and surveys evolving technologies for lesion formation (Table 120-1). The interested reader is encouraged to review the chapter in the previous edition of this textbook for older references in the field. The first catheter-based method of ablating cardiac tissue—high-energy direct current (DC) shock—was reported in 1982. With an external defibrillator used as the power source, DC shocks were successfully applied via a transvenous electrode catheter positioned adjacent to the His bundle to ablate the atrioventricular (AV) node.1,2 Although effective, this procedure caused significant collateral damage, resulting in complications including cardiac perforation and tamponade, with in-hospital mortality rates approaching 6%.3 It became clear that for catheter-based ablation to gain wider application as a therapeutic technique, safer methods to create ablative lesions were needed. The application of radiofrequency (RF) ablation to the treatment of cardiac arrhythmias was first reported by Huang in 19854 and quickly replaced DC ablation as a safer and more effective alternative.5 RF ablation was rapidly adopted in the treatment of multiple arrhythmias including Wolff-Parkinson-White (WPW) syndrome,6 supraventricular tachycardia (SVT),7 atrial flutter,8 and ventricular tachycardia (VT).9 RF energy is typically delivered between a unipolar electrode at the tip of the ablation catheter and a large dispersive grounding patch on the patient’s skin, typically on the abdomen or thigh. The passage of RF current from the ablation catheter through tissues to the grounding patch results in resistive tissue heating and lesion formation. Because the surface area of the ablation catheter tip is small compared with the dispersive patch, current density is high in the immediate vicinity of the catheter tip. This results in resistive heating at the catheter tip–tissue interface and a small rim of surrounding tissue. Deeper ablative lesions result from conductive heating of surrounding tissues. Significant tissue heating during RF delivery is usually associated with impedance drops of 5 to 10 Ω.10 Electromagnetic energy sources from different parts of the frequency spectrum have been used for ablation of cardiac arrhythmias (Figure 120-1). Typically, electromagnetic energy is delivered via catheter to targeted tissue until it is adequately heated to cause irreversible thermal damage. The RF spectrum spans a wide range of frequencies from 3 kHz to 300 GHz. RF generators typically deliver unmodulated sine wave alternating current (AC) at frequencies between 500 and 1000 kHz, which oscillates too rapidly to depolarize myocardium and induce arrhythmias. Figure 120-1 The electromagnetic spectrum as displayed is a continuum of electromagnetic waves arranged according to frequency and wavelength. In catheter ablation, electromagnetic energy is delivered to the myocardium via transvenous catheters to create thermal lesions. Various energy sources have been used for catheter ablation of cardiac arrhythmias. Radiofrequency (RF) energy is currently the most commonly used energy source in catheter ablation of cardiac arrhythmias. Microwave energy has been investigated as another potential energy source for creating myocardial lesions. EUV, Extreme ultraviolet; FUV, far ultraviolet; HF, high frequency; LF, low frequency; MF, mid frequency; UHF, ultrahigh frequency; UVIS, ultraviolet imaging spectrum; VHF, very high frequency. The heat generated per unit volume of tissue is inversely related to the fourth power of the distance from the catheter tip. Therefore, doubling the distance from the catheter tip reduces resistive heating by 94%. Because of the rapid reduction in heating with distance, lesions created by RF energy are typically small and well circumscribed.11 Only a 1- to 2-mm rim of tissue directly adjacent to the catheter tip is heated resistively; deeper tissues are heated by passive thermal conduction. This conductive heating is responsible for most of the lesion volume from RF ablation catheters (Figure 120-2). Figure 120-2 A, Currently available radiofrequency (RF) ablation catheter designs. Lesion depth has increased with larger catheter tips and methods for cooling the ablation electrode (irrigation). The ability to sense contact pressure and deliver RF via multiple electrodes in a bipolar configuration is a recent development. B, Lesion formation during RF delivery on the epicardial (arrow) surface of a Langendorff-perfused rabbit heart. This heart was imaged using a 30-MHz ultrasound system (VisualSonics, Toronto, Canada). (Courtesy Aman Mahajan, MD, PhD, University of California at Los Angeles.) Experimental studies have shown that irreversible tissue destruction occurs when tissue temperature exceeds 50° C.11 The radius of the 50° C isotherm in the tissue, which effectively corresponds to the lesion boundary, increases in direct proportion to the temperature of the source, up to catheter tip–tissue interface temperatures of around 100° C. At higher temperatures, plasma boils, resulting in coagulum or char on the catheter tip, potential embolization, abrupt impedance rise, reduced current delivery, and ineffective tissue heating. To avoid excessive heating, RF ablation catheters incorporate thermocouples or thermistors to allow real-time monitoring of catheter tip temperature during ablation. With this feedback, RF generators titrate power to maintain a specified catheter tip temperature when set to temperature-guided mode. RF generators can also deliver energy in power-guided mode, in which the operator manually sets power. Although current technology does not allow direct measurement of myocardial tissue temperature, surrogate markers of tissue temperature and tissue destruction during RF ablation include impedance drop, electrogram amplitude reduction, and loss of excitability with pacing (Box 120-1). With standard nonirrigated RF ablation catheters, the power output of the RF generator is adjusted manually or automatically to achieve a temperature of 50° C to 70° C at the electrode–tissue interface. Catheter–tissue contact is a key determinant of lesion formation with RF catheter ablation (Box 120-2). If the catheter is not in direct contact with myocardial tissue, virtually all energy will be dissipated in the blood pool without effective tissue heating. Operators rely on multiple sources of information to assess catheter–tissue contact, including tactile feedback, fluoroscopic catheter movement, intracardiac echocardiography, catheter tip impedance, unipolar injury current, and electrogram variability from beat to beat. However, even with all of these data, a significant discrepancy is noted between estimated and actual tissue contact force.12 This motivated the development of ablation catheters, currently being tested in the United States and commercially available in other countries, which incorporate a fiberoptic sensor in the ablation catheter tip to estimate contact force and catheter orientation (TactiCath, Endosense, Meyrin, Switzerland).13 Initial studies have shown that lesions are larger and steam pops more likely with greater contact force.14 Higher contact force, especially during RF delivery, also increases the risk of cardiac chamber perforation.15 Eventually, such catheters may improve both efficacy and safety of RF catheter ablation by helping the operator to achieve sufficient but not excessive catheter–tissue contact.16,17 A typical 7 French diameter RF ablation catheter with a 4-mm-long ablation electrode tip creates a lesion 5 to 6 mm in diameter and 2 to 3 mm deep. RF ablation catheters with 8- to 10-mm tips expose a greater surface area of the ablation electrode to the blood pool, increasing convective cooling at any given level of blood flow and creating a larger tissue–catheter tip interface, which results in larger lesions (see Figure 120-2). An alternative to the conventional platinum-iridium RF ablation catheter tip is the gold-tip catheter, which provides the advantage of much higher thermal conductivity and allows greater power delivery without excessive heating of the catheter–tissue interface.18 Although in theory, this should translate into more effective lesion formation, clinical studies have not demonstrated increased efficacy or safety in treatment of typical right atrial flutter,19 AV nodal reentry tachycardia,20 and atrial fibrillation.21 In the future, other compounds or alloys may prove superior to platinum-iridium for catheter tip construction. One challenge of using RF as the energy source for myocardial lesion formation is lack of information about true tissue temperature. Another issue is lag time of tissue injury after RF application; tissue temperature can continue to rise after termination of RF delivery, potentially resulting in lesion growth and collateral damage. Challenges have also arisen during RF energy delivery to the epicardial surface of the heart. Standard RF catheters reach high temperatures quickly in this confined space without cooling blood flow.22 A challenge for all ablation technologies is creating permanent transmural lesions. Non-transmural lesions may be associated with acute conduction block due to edema or transient tissue injury but recovery of conduction and recurrence of arrhythmia after the ablation procedure. It can be difficult with standard RF catheters to create contiguous or overlapping lesions; this becomes more significant for longer lines of ablation. Gaps in lesion lines can be proarrhythmic, acting as a zone of slow conduction and facilitating reentry (Figure 120-3).23 Figure 120-3 Optical Mapping Images of Conduction Through Straight and Bifurcated Gap Preparations Newer catheters have been designed to address some of the special challenges encountered during RF ablation. Linear catheters with multiple electrodes, which can ablate sequentially or simultaneously, have been developed with the goal of creating more continuous linear lesions without gaps.24,25 Bipolar RF catheters deliver current between two adjacent electrodes, and duty-cycled phased RF systems alternate between unipolar and bipolar energy delivery.26,27
Catheter Ablation
Technical Aspects
Historical Context of Catheter Ablation
Radiofrequency Ablation
Radiofrequency Energy Sources
Heat Transfer to Tissue
Limitations of Standard Catheter-Based Radiofrequency Ablation
Radiofrequency (RF) lesions are denoted by broken circular lines; solid straight lines indicate the positions of incisions from the tissue edges to the RF lesions. The top panels demonstrate that the orange wave fronts of depolarization have propagated from the pacing sites (denoted with asterisks) to the openings of the gaps in RF lesions. The lower panels demonstrate that the orange wave fronts have emerged through both straight and bifurcated gaps after 4 and 8 ms, respectively. Note that the bifurcated gap has resulted in the formation of two wave fronts from the two arms of the bifurcated gap. (Reproduced with permission from Perez FJ, Wood MA, Schubert CM: Effects of gap geometry on conduction through discontinuous radiofrequency lesions. Circulation 113:1723-1729, 2006.)
Recent Developments in Radiofrequency Ablation
Stay updated, free articles. Join our Telegram channel
Full access? Get Clinical Tree
Catheter Ablation: Technical Aspects
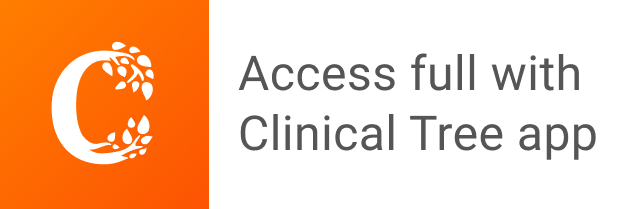