Cardiovascular Physiology
Daniel J. Penny
Ronald A. Bronicki
Introduction
The concepts which underlie our understanding of cardiovascular physiology have evolved significantly in recent years. This chapter aims to summarize some of the current concepts related to these key physiologic processes.
One framework which might be usefully applied to the study of cardiovascular physiology would be one in which a ventricle, primed by adequate venous return generates “pressure work.” This is converted into “flow work” in the aorta and the pulmonary trunk. Flow within these great arteries is then distributed between vascular beds in order to optimize metabolism, as in the case of the systemic circulation, or oxygenation, as in the pulmonary circulation.
This chapter will consider some concepts related to ventricular function and complement some of the concepts outlined in other chapters in this textbook. It will examine how pressure-work within the ventricle is converted into flow-work within the great arteries, the concept of ventriculovascular coupling. It will explore the distribution of flow within and between vascular beds in the systemic circulation and the determinants of flow in the pulmonary vascular bed. It will then consider how cardiovascular performance relates to systemic metabolism. Finally, as there are significant developmental changes in all aspects of cardiovascular physiology, it will examine the fetal, neonatal, and transitional circulation.
Ventricular Function
The accurate description of the function of the heart, its metabolic demands, as well as its coupling to the vasculature is fundamental to our understanding of normal and abnormal cardiovascular physiology. In routine clinical practice we are often limited to the estimation of ventricular systolic and end-diastolic pressure and some measure of volume change (e.g., fractional shortening or ejection fraction), although recently newer echocardiographic and magnetic resonance indices (which are considered elsewhere in this textbook) have provided new insights.
The classic work of Wiggers (1), which examined the temporal changes in ventricular pressure and volume during the cardiac cycle remains as important to our understanding of ventricular function today as when it was originally formulated. For simplicity, this section divides the cardiac cycle into “phases” based on the model of Wiggers, but it must be recognized that this separation is somewhat an oversimplification as there is considerable overlap between the different periods and the underlying physiologic phenomena that are occurring within the ventricle during them. In addition, there is often incoordination of function within the heart, particularly in disease.
Briefly, with the onset of ventricular depolarization, ventricular pressure rapidly increases initially above left atrial pressure, until it approaches the level of aortic diastolic pressure. As both the mitral valves and aortic valves are closed during this time, this is labeled the “isovolumic contraction period.” Given that the major change within the ventricle during this period is pressure development, measures of ventricular function have been based on the rate with which the ventricle develops pressure during isovolumic contraction. These include the maximum rate of increase in pressure (dP/dtMAX) or one of its derivatives, derived to reduce its sensitivity, particularly to preload (2).
A further increase in ventricular pressure above either aortic arterial pressure (in the case of the left ventricle) or pulmonary arterial pressure (as in the case of the right ventricle) marks the onset of ventricular ejection. The efficiency of ventricular ejection will be determined by the function of the ventricle; thus, several ejection phase markers of ventricular performance have been utilized, for example, fractional shortening and ejection fraction. Derived measures have been developed, again to account for alterations in ventricular loading, for example, the relationship between stroke volume and end-systolic stress (afterload) or between the velocity of circumferential shortening (VCFc) and end-systolic stress (3).
The onset of diastole is usually considered to be at the time when ventricular pressure falls below aortic or pulmonary arterial pressure and the semilunar valves close, although clearly the onset of ventricular pressure decay (relaxation) occurs before this time. Furthermore, because of differences in the loading conditions imposed on the right and left ventricles, there is typically a considerable “lag phase” during which the pulmonary valve remains open, even though right ventricular pressure is significantly less than pressure in the pulmonary artery (4). Given that pressure decay is the main phenomenon occurring in the ventricle during this time, a number of measures of ventricular diastolic function have been developed, based on the rate of pressure decline. These include the maximum rate of pressure decay (dP/dtMIN) or related measures, derived in order to reduce the impact of load and heart rate (5).
Once pressure within the ventricle has decayed to below that in the atrium, the atrioventricular valve opens and the ventricle begins to fill. The early phase of ventricular filling is principally determined by the continued rate of pressure decay within the ventricle, while later in diastole, the most important determinants of filling are the compliance or elastic characteristics of the ventricle; thus, additional measures of ventricular diastolic function are based on these filling patterns (see Chapter 13).
Above and beyond the temporal changes in ventricular pressure and volume, further important insights into not only the function of the ventricle, but also into the efficiency with which the ventricle is coupled to the vasculature can be gained from a consideration of the time-varying relationship between ventricular pressure and volume, represented by the ventricular pressure–volume loop. Interest in the use of the pressure–volume loop has increased since the development of the conductance catheter. Examination of the pressure–volume relationship provides an enormous amount of information about the systolic and diastolic function of the ventricle, as well as the coupling between the ventricle and the vasculature (6). Although detailed consideration of the ventricular pressure–volume loop is beyond the scope of this chapter, several observations are important for the purposes of this chapter (Fig 8.1). First, the end-systolic pressure volume points (the upper left-hand corner of the loop) at a constant level of inotropy appear to adopt a linear relationship as ventricular volume is altered. Indeed this linear relationship appears to be present at all times during the cardiac cycle, such that cardiac contraction
could be considered as a “time-varying elastance” (Fig. 8.1). This elastance appears to be maximal around the time of end-systole (EMAX). Second, the relationship between ventricular pressure and volume during diastole provides measures of ventricular compliance (the end-diastolic pressure–volume relationship, EDPVR) (Fig. 8.1). Third, the ratio of end-systolic pressure to stroke volume is constant under any steady state of vascular load, so that the properties of the vasculature can be condensed into a single measure, the so-called effective arterial elastance (Ea).
could be considered as a “time-varying elastance” (Fig. 8.1). This elastance appears to be maximal around the time of end-systole (EMAX). Second, the relationship between ventricular pressure and volume during diastole provides measures of ventricular compliance (the end-diastolic pressure–volume relationship, EDPVR) (Fig. 8.1). Third, the ratio of end-systolic pressure to stroke volume is constant under any steady state of vascular load, so that the properties of the vasculature can be condensed into a single measure, the so-called effective arterial elastance (Ea).
The Translation of Ventricular Pressure-Work to Flow-Work in the Great Arteries
Once the ventricle generates pressure-work and the semilunar valves open, it is then necessary to translate the ventricular pressure-work into flow in the pulmonary trunk and the aorta. The efficiency with which this translation occurs is largely determined by the properties of the vasculature. The traditional measures of vascular function, which are widely used in routine clinical practice are the systemic and pulmonary vascular resistances. While useful for prognosis and clinical management, these measures are based on a model of the circulation in which a constant pressure source (the ventricle) is coupled to a rigid “pipe.” In reality, the situation is considerably more complex. As discussed, the ventricle is a complex time-varying source of elastance and far from being single rigid pipes, the systemic and pulmonary vasculatures consist of compliant vessels with complex impedances, resistances, and sources of energy reflection, all of which will impact on the ventricle during ejection (7).
There is growing interest in the study of the coupling between the ventricle and the vasculature, given that it is now appreciated that the nature of this coupling is a fundamental determinant of performance in a variety of disease states. However, there are a number of challenges in gaining these insights, the least of which is to design a robust model of coupling, which can not only characterize the properties of both the ventricle and the vasculature, but also predict the changes in integrated function under varying physiologic and pathologic conditions.
It is generally agreed that due to the complex characteristics of the vascular bed and the pulsatile nature of arterial flow, the load imposed by the vasculature is most completely characterized in the form of impedance spectra in the frequency domain. Given that measurement of vascular impedance reflects the properties of the whole vascular bed distal to a point of measurement, the derivation of ascending aortic input impedance or pulmonary arterial input impedance provides the most complete assessment of the hydraulic load faced by the left or right ventricles respectively. Despite its advantages many challenges exist in using impedance in coupling models, principally related to its intrinsic complexity. Furthermore, given that it is derived in the frequency domain while most indices of ventricular function are derived in the time-domain, it is difficult to directly integrate vascular and ventricular properties within a single model without oversimplification (8).
An alternative approach is based on the pressure–volume relations (9). In this framework the vascular properties are condensed into a single measure of effective arterial elastance (Ea) which predicts that the ratio of end-systolic pressure (Pes) to stroke volume is constant under a given steady state impedance load. It is simple to use this model to examine the relationship between arterial and ventricular elastance (EMAX), in examining the integration of ventricular and vascular properties and the changes in the level of integration during physiologic and pathologic processes. For example, it has been observed that in normal subjects with an ejection fraction of 60% or more, the ventricular elastance is typically nearly double the arterial elastance. This relationship affords an optimal coupling between ventricular work and myocardial O2 consumption. In patients with ejection fractions of 40% to 50%, as in the case of moderate cardiac failure, arterial and ventricular elastances become nearly equal, which provide an optimal stroke work at a given end-diastolic volume (but at a lesser efficiency of O2 utilization). As ventricular failure progresses and ejection fraction declines further, ventricular elastance is typically less than arterial which provides suboptimal coupling between ventricular work and both O2 consumption and stroke volume. It therefore
appears that under normal circumstances, the coupling between the ventricle and vasculature is such that metabolic efficiency (relationship between work and oxygen consumption) is maximized; as cardiac function declines, this relationship is subordinated by an attempt to maintain stroke work in the face of declining ventricular function. As ventricular function declines further and compensation mechanisms are overwhelmed the ventricle and vasculature become uncoupled to the detriment of both stroke work and metabolic efficiency (10).
appears that under normal circumstances, the coupling between the ventricle and vasculature is such that metabolic efficiency (relationship between work and oxygen consumption) is maximized; as cardiac function declines, this relationship is subordinated by an attempt to maintain stroke work in the face of declining ventricular function. As ventricular function declines further and compensation mechanisms are overwhelmed the ventricle and vasculature become uncoupled to the detriment of both stroke work and metabolic efficiency (10).
An alternative time-based framework for the assessment of ventriculovascular coupling, has been developed by Parker and coworkers, the so-called method of characteristics (11). This formulation considers that the heart transfers energy into the circulation in the form of forward travelling waves (compression or expansion), which modulate flow and pressure in the vasculature. The vasculature, in turn, is a source of waves (e.g., from bifurcations and the microvasculature) that travel backward toward the heart. The interactions between these forward- and backward-running waves result in a complex pattern of pressure and flow augmentation at different times during the cardiac cycle and at different points in the circulation. Insights into the power of these waves can be obtained by mathematical transformation in the time-domain of pressure and flow signals. This model has been applied to examine the regulation of flow in the fetal pulmonary circulation (12), for example, and to examine ventriculovascular coupling in patients after reconstruction of the aortic arch (13).
Control of Flow in the Systemic Circulation
Once blood enters the aorta it is distributed through a large number of vascular beds and the properties of each receiving a number of control inputs. These control mechanisms are determined both centrally through neural and hormonal pathways, as well as locally through metabolic and autoregulatory mechanisms. This section will first consider some of these control mechanisms and then examine the degree to which they may influence regional flow within some of the more important vascular beds.
Central Neural Control
Neural control is particularly important in the instantaneous regulation of arterial pressure and in the rapid changes which occur in regional flow during times of acute stress. This rapid regulation of flow by neural mechanisms is effected through reflex circuits, composed of an afferent limb, which transmits information about the physiologic state to an integrating site within the medulla oblongata, which, by modulating activity within the efferent limb of the autonomic nervous system mediates changes in vascular tone.
The most powerful afferent limb of neural control originates within mechanoreceptors in the carotid sinuses and aortic arch, which respond to changes in arterial pressure (baroreceptors). At least two types of baroreceptors have been identified, with the first controlling dynamic changes in blood pressure and the second being responsible for control of resting blood pressure (14). Alterations in tension within these receptors modulate nerve impulses to the cardioregulatory and vasomotor centers of the medulla oblongata, which regulate the output in the efferent limb of the reflex (below). As a result, an increase in arterial pressure by stimulating the carotid sinus results in slowing of the heart rate, vasodilation, and a restoration of arterial pressure. These baroreceptors themselves are innervated by efferent fibers of the sympathetic nervous system, which suggests that sympathetic activity may modify the “gain” of the baroreceptor responses. Given the profound influence of the carotid sinus baroreceptors on arterial pressure, there is increasing interest in the potential for chronic carotid sinus stimulation to treat resistant systemic hypertension (15).
Mechanoreceptors are also found in the walls of the atria and ventricles. They are located in the walls of both atria at the venoatrial junctions (16), and are scattered throughout the left ventricle and interventricular septum. Two kinds of atrial receptors have been described. Type A receptors fire during atrial contraction and respond to changes in atrial pressure, and type B receptors fire during ventricular systole and respond to changes in atrial volume (17). In particular, atrial receptors modulate the sympathetic activity to the renal vasculature (14), which combined with their influences on hormonal function (below) mediates their profound influence on intravascular volume. Two different types of mechanoreceptors are found in ventricular myocardium. The first fire in a pulsatile manner in time with cardiac rhythm and are small in number. The second respond to mechanical stimulation and to various drugs and chemicals through nonmyelinated afferent nerves known as C fibers. Stimulation of C fibers, which are primarily located in the left ventricle results in hypotension and bradycardia through parasympathetic stimulation and sympathetic inhibition (16).
Significant additional afferent inputs into the neural control of the circulation come from chemoreceptors, which are primarily located in the carotid body, aortic arch, and brain, as well as in coronary vessels, muscle, and lung. It appears that under normoxemic and normocapneic conditions, they exert little effect, but they are important in modulating the cardiorespiratory response to hypoxemia and hypercapnia.
The most important central integrating site for these neural inputs for control of the circulation is in the medulla oblongata. The activity of these medullary centers may be modified by other centers within the brain, in particular, the hypothalamus.
Central mechanisms in the medulla regulate the output of the sympathetic and parasympathetic neural systems, the efferent limb of neural control of the circulation. The primary efferent effectors are the sympathetic vasoconstrictor fibers, which when stimulated release norepinephrine from their nerve-endings. Other substances are also released, including monoamines, polypeptides, purines, and amino acids, some of which have direct vasoactive effects while others modulate the release, actions, and reuptake of norepinephrine (14). Impulses carried through vasoconstrictor fibers contribute to the normal vascular tone or baseline constriction that is present at rest in most vascular beds; thus they are the main mechanism for regulating blood pressure in the unstressed state. These vasoconstrictor fibers are prevalent in skeletal muscles, where intrinsic tone is fairly high under resting conditions. They are much less prevalent in the cerebral and coronary beds. Sympathetically mediated venoconstriction modulates venous capacitance, and in turn, circulating volume.
Sympathetic stimulation may also mediate vasodilation. The transmitter in vasodilator fibers is thought to be acetylcholine, although in primates it may be epinephrine. These vasodilator fibers may cause a small anticipatory increase of blood flow to the skeletal muscle. However, once muscle exercise begins, local vasodilation probably plays a more important role (18).
The parasympathetic system primarily controls heart function and rate although it does have a limited role in control of the peripheral circulation, through the release of acetylcholine. Parasympathetic vasodilator fibers are found in the cerebral and myocardial circulations and in the bladder, rectum, and external genitalia.
Central Hormonal Control
An additional potent regulation of vascular function is provided by circulating levels of vasoactive hormone, including catecholamines, angiotensin II, vasopressin, eicosanoids, nitric oxide (NO), and peptides.
Vascular smooth muscle is abundant with receptors for catecholamines. These receptors are responsive to both locally produced catecholamines, originating from the local sympathetic innervation, endogenous circulating catecholamines from the adrenal, as well as to exogenous sympathomimetic drugs. Stimulation of the so-called α-adrenergic receptors results in vasoconstriction, while stimulation of β-adrenergic receptors causes vasodilation.
A powerful vasoconstrictor response may be initiated through the actions of angiotensin II, produced by the renin–angiotensin–aldosterone system. The juxtaglomerular apparatus in the kidney secretes renin in response to decreased renal arterial pressure or a decrease in extracellular fluid volume. Renin, in turn, cleaves angiotensinogen to angiotensin I, which is then converted to angiotensin II by a converting enzyme found particularly in the lung and vascular endothelium. As well as direct vasoconstrictor properties, angiotensin II also mediates vasoconstriction through its action within the medulla oblongata by stimulating the hypothalamus to synthesize antidiuretic hormone (vasopressin).
Another group of hormones that participate in regulation of the systemic circulation are the natriuretic peptides. At least three main types of natriuretic peptide have been identified: Atrial natriuretic peptide (ANP), B-type natriuretic peptide (BNP), and C-type natriuretic peptide (CNP). ANP is released from myocytes mainly within the atria, usually in response to stretch, but is also released in small amounts by ventricular myocytes. ANP has vasodilator and cardioinhibitory effects, and in the kidney, reduces the tubular reabsorption of sodium (19). Circulating levels of ANP are increased in certain diseases including congenital heart disease associated with elevated atrial pressures, congestive heart failure, valve disease, hypertension, coronary artery occlusion, and atrial arrhythmias.
BNP is secreted by the ventricles, as well as by the atria in response to volume expansion and pressure overload and has similar circulating actions to ANP. BNP has received increasing attention as a circulating biomarker for a number of cardiovascular conditions. CNP, by contrast acts more locally, being stored in endothelial cells, particularly in the coronary and mesenteric circulations and mediating vasodilation through its actions on Gi proteins and in turn, adenylyl cyclase as well as phospholipase-C (20).
Local Control by the Endothelium
The vascular endothelium plays a key role in regulating vascular tone, by producing a variety of substances which mediate either relaxation (vasodilation) or contraction (vasoconstriction) of the underlying vascular smooth muscle (21).
The endothelial-derived vasodilator substances, which include NO, prostacyclin (PGI2), adenosine, and the so-called endothelial-derived hyperpolarizing factor (EDHF) have been reviewed elsewhere in this textbook. Briefly NO mediates vasorelaxation through its action of soluble guanylate cyclase, with resulting formation of the vasodilator cGMP, while PGI2 appears to act predominantly through its actions of cAMP. The structure of EDHF is not yet determined, although it may be closely related to CNP and promotes vasorelaxation by inducing hyperpolarization of vascular smooth muscle cells (21) through activation of K+ channels. Several vasoconstrictor substances are also produced by the endothelium and again have been reviewed elsewhere. These include endothelin (ET)-1, which exerts complex vasoactive influences depending on the site and type of receptor upon which it acts, as well as angiotensin II. More recently, the role of the very potent mediator urotensin, which again can mediate both vasoconstriction and vasodilation, is receiving attention (22).
Local Control by Metabolism
Flow to many tissues of the body is regulated by changes in local metabolic demand. It appears that the “switch,” which couples increases in metabolism to local vasodilation resides in metabolism-related changes within the local chemical microenvironment. For example, a decrease in pO2, an increase in pCO2, or an increase in H+ or K+ concentration each causes arteriolar vasodilation independent of endothelial function. Many cells other than endothelial cells will release the potent vasodilator adenosine, in response to increased metabolism or decreased oxygen tension.
Local Control by Red Blood Cells
There is increasing interest in the regulation of local flow by red blood cells (23). This mechanism is closely related to metabolic control, in that it appears that deoxygenation of hemoglobin results in the release of a number local endothelial-dependent and endothelial-independent vasodilators, including adenosine triphosphate (ATP) and NO (24).
Local Control by Autoregulation
The ability for local flow to remain relatively constant over a range of arterial input pressures is particularly important for organs which cannot significantly alter their metabolic requirements in the face of hypotension. This phenomenon is termed “autoregulation.” The primary mechanisms for autoregulation remain unclear and appear to differ between vascular beds. Potential mechanisms include an intrinsic myogenic response within the vascular smooth muscle, which allows the arterioles to constrict via an endothelial-independent mechanism in response to changes in transmural pressure. This possibly occurs through the interactions of cell surface integrins with extracellular matrix proteins and alterations in calcium currents (25). Alterations in the production of local metabolic factors, changes in sympathetic tone, and in the kidney, “tubular glomerular feedback” (below) are also likely to contribute.
Flow to Specific Vascular Beds
The dynamic interplay between the different mechanisms described above, mediate the variability in flow within and between the different regional vascular beds. As a general principle, the metabolically highly active organs such as the brain and heart are primarily regulated by local mechanisms, whereas the less active organs are more subject to central neural and hormonal controls. Specialized beds such as the renal and hepatic circulations, which receive blood for unique activities such as metabolic degradation and excretion, hematopoiesis, and blood pressure control, have unique combinations of control mechanisms. The myocardium is not discussed here, but is considered elsewhere in this textbook.
The Cerebral Circulation
The cerebral circulation has been the most extensively studied and characterized. It is unique in a number of respects. First, there is a blood–brain barrier created by a continuous lining of endothelial cells linked by tight junctions, which provides some resistance to changes in concentrations of various circulating constituents such as H+ and catecholamines. Second, a significant component of the cerebral vascular resistance is formed by the large arteries, which appear to respond in a similar fashion to the arterioles in response to stresses such as hypoxia. Third, the cerebral circulation is encased in a closed box, the skull, so that tissue pressure is an important determinant of flow. Finally, there is great heterogeneity in blood flow to different parts of the brain and this heterogeneity may change during development.
Due to the high metabolic rate of cerebral tissue and the limited capacity for storage of substrate, cerebral flow must be precisely regulated if nutrient and oxygen supply is to be maintained.
Cerebral perfusion is highly sensitive to changes in arterial CO2 tension, with an approximate increase of 3% to 6% in flow occurring for every mm Hg change in PCO2 above eupneic levels (26). The cerebrovascular bed is also sensitive to hypoxia, although at least in the mature brain, cerebral flow is usually unchanged by reductions in PO2 to around 50 mm Hg. It does appear that the fetal cerebrovascular bed is likely to be significantly more sensitive to oxygen (27).
Cerebral perfusion is highly sensitive to changes in arterial CO2 tension, with an approximate increase of 3% to 6% in flow occurring for every mm Hg change in PCO2 above eupneic levels (26). The cerebrovascular bed is also sensitive to hypoxia, although at least in the mature brain, cerebral flow is usually unchanged by reductions in PO2 to around 50 mm Hg. It does appear that the fetal cerebrovascular bed is likely to be significantly more sensitive to oxygen (27).
The central role for autoregulation in the regulation of cerebral flow has been underscored since 1959 when Lassen published a review article (28), which presented a plot of average blood pressure and cerebral flow from multiple studies during a range of drug- or disease-related changes in arterial pressure. The plot revealed the now classic relationship wherein flow appeared to be completely stable across a relatively wide range of pressures. While this concept is still considered to be valid and while it has been relatively consistently demonstrated in isolated tissues, there are few data which characterize the normal within-subject relationship between arterial pressure and flow, principally because there are several challenges in developing the relationship (29). First the normal baroreflex function limits the range of pressures that can be studied. Second, pharmacologic agents, which change arterial pressure may in themselves alter the resistance vessels of the brain. Third, alterations in sympathetic tone, which may occur during hypotension may also influence cerebral flow, as it is known that the cerebrovascular bed is extensively innervated by adrenergic and cholinergic fibers. Patient studies have demonstrated that cerebral flow is generally increased by cervical ganglionectomy, suggesting an important modulation of cerebral flow from the autonomic nervous system (26).
Renal Circulation
The renal circulation has a number of unique features, including its extremely high flow because of the requirements of glomerular ultrafiltration, as well as the presence of two distinct capillary beds to allow filtration and reabsorption.
Flow to the adult kidney represents up to 25% of cardiac output. Most of this flow courses via the afferent arterioles in the renal cortex to the glomerular capillary bed under relatively high pressure to allow a large production of ultrafiltrate. Distally, an efferent arteriolar system decreases hydrostatic pressure to low levels, which in addition to osmotic forces promotes the reuptake of the filtrate. There is considerable variability of flow within the renal cortex itself. The outer cortex receives a relatively small proportion of flow and is composed of small glomeruli with low single-nephron glomerular filtration. The inner or juxtaglomerular cortex receives far more flow per weight and is composed of very large glomeruli with high filtration rates. The renal medulla is composed of the outer medulla (the descending and thick ascending limbs of the loops of Henle and collecting duct segments) and the inner medulla (thin segments of the loops of Henle and the terminal portions of the collecting system). The inner medulla is perfused by the vasa recta and receives the least flow per weight and at very slow transit times, which is critical to the reuptake of ultrafiltrate.
Under normal conditions, a key mechanism for the regulation of flow to the renal cortex appears to be autoregulation (24). It appears that at least two mechanisms underpin this local regulation of renal flow. The first of these is the aforementioned intrinsic myogenic response within the vascular smooth muscle. The second unique mechanism contributing to autoregulation of renal flow is the phenomenon of tubular glomerular feedback, whereby alterations in the concentration of sodium in the tubular fluid reaching the macula densa of the distal nephron acts to alter the diameter of the juxtaposed afferent arteriole (23). Renal autoregulation may be perturbed in a number of disease states (30).
There is increasing interest in the role of the renin–angiotensin system in the regulation of renal function and hemodynamics (31). In addition to important vasoregulatory functions within the kidney, angiotensin II modulates tubular transport. Further, given that all components of the renin–angiotensin system are expressed within the kidney itself, the importance of local mechanisms is being increasingly studied. The effects of angiotensin II on renal hemodynamics are complex. First, angiotensin II has direct vasoconstrictor actions. Second, this vasoconstrictor response is highly modulated by NO synthesis, the expression of which is increased by angiotensin II. As a result, angiotensin II-mediated increases in the local production of NO counteract the vasoconstrictor effects, particularly in the afferent arteriole. Indeed, under some circumstances, large doses of exogenous angiotensin II have been demonstrated to increase renal flow through a NO-mediated mechanism. Under circumstances of reduced NO bioavailability, for example in oxidative stress, the vasoconstrictor actions of angiotensin II are exaggerated (32,33). Third, angiotensin II has additional vasoactive effects by augmenting tubular glomerular feedback (31).
Locally produced endothelin plays a crucial role in renal control. Every cell type in the kidney can synthesize endothelin and contain abundant endothelin receptors, particularly the vasculature and the medulla. As a result, endothelin not only modulates total and regional renal flow, but also glomerular filtration, Na and H2O handling, and acid–base regulation. The kidney, particularly the medulla is particularly sensitive to the vascular effects of endothelin, compared to other vascular beds and endothelin-mediated vasoconstriction appears to be mediated through activation of ETA and ETB receptors (34). Alterations in the endothelin pathway appear to be central in the pathophysiology of several acute and chronic renal conditions (35).
During stress, however, autonomic rather than autoregulatory mechanisms predominate and act primarily to limit renal blood flow. Both α1– and α2-adrenergic receptors are present in the kidney and stimulation of both by neural discharge or circulating catecholamines causes renal vasoconstriction, redistributing blood away from the kidney as blood is distributed away from the peripheral circulation. Dopaminergic and β-adrenergic receptors are also present. Stimulation of both causes vasodilation.
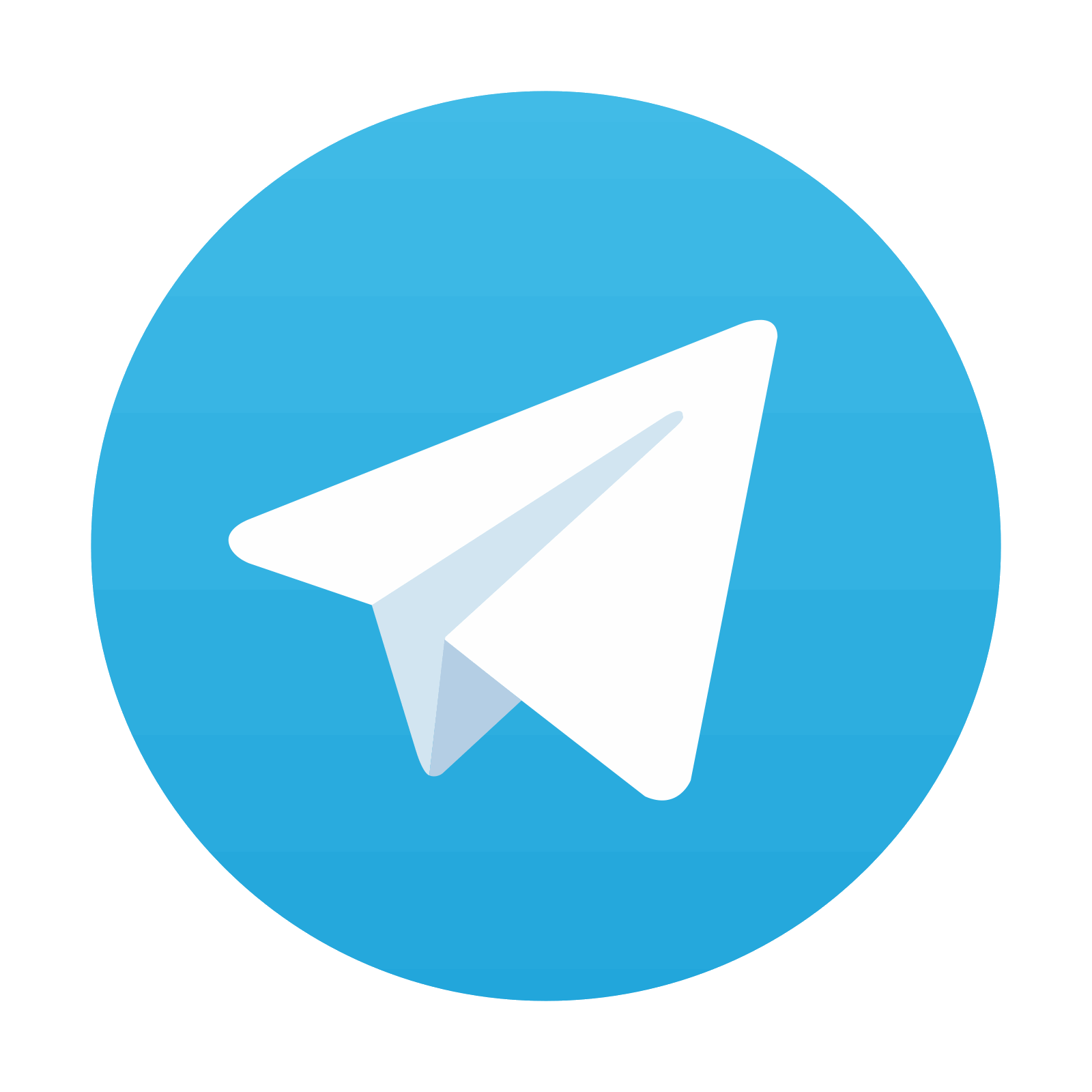
Stay updated, free articles. Join our Telegram channel
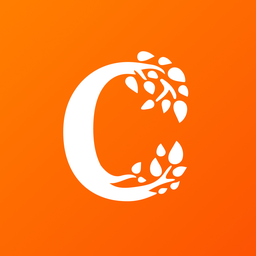
Full access? Get Clinical Tree
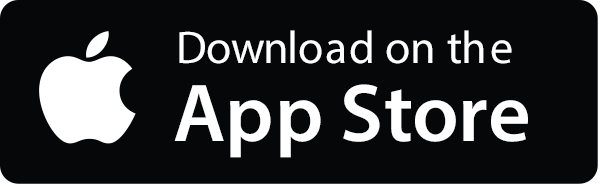
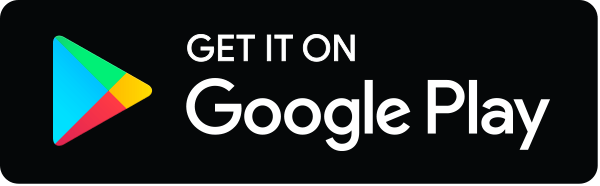
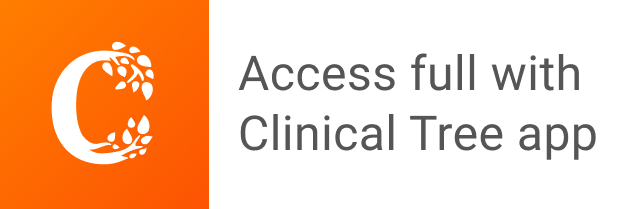