Cardiovascular Magnetic Resonance Imaging
Milind Y. Desai
Richard D. White
David A. Bluemke
Joao A. C. Lima
Introduction
Cardiovascular magnetic resonance imaging (MRI) is a rapidly evolving technology that is very well suited for the morphologic and physiologic evaluation of a wide range of acquired and congenital disease processes affecting the heart, pericardium, and great arteries and veins of the thorax. MRI is unique in its ability to provide four-dimensional (three spatial dimensions over the dimension of time) imaging of the cardiovascular system based on definition of high-detail anatomy, histologic characterization, intracardiac or intravascular blood flow, cardiac chamber contraction and filling, regional myocardial mechanics, and tissue perfusion. Hence, MRI is playing a major role as a diagnostic modality in all facets of cardiovascular medicine. Furthermore, its potential is clearly becoming evident in the fields of atherosclerosis imaging, molecular imaging, and interventional cardiovascular medicine.
Principles and Techniques
Brief History
Nuclear magnetic resonance is a phenomenon exhibited by certain atomic nuclei. It was introduced by Block et al. (1) and Purcell et al. (2) in 1946. In 1973, Lauterbur (3) first described a method for producing images using related techniques, and as early as 1977 human whole-body images were already being produced by MRI (4). By the early 1980s (5,6), high-quality static anatomic imaging of the cardiovascular structures was being accomplished by electrocardiogram (ECG)-gated MRI. The mid to late 1980s saw the development and clinical implementation of dynamic MRI techniques allowing physiologic assessment of cardiac function and blood flow (7,8); this was promoted by the use of the more rapid acquisition techniques (9). Since then, MRI has profited from technical advances allowing the following capabilities: near-real-time imaging (10,11), tissue tagging-tracking (12), and three-dimensional (3D) acquisition and display (13); the result has been improvement in the assessment of myocardial physiology and complex and/or fine-detail anatomy of the cardiovascular system.
Magnetic Resonance Imaging Principles
A detailed discussion of MRI principles is beyond the scope of this book. A brief discussion follows. Atomic nuclei with odd numbers of protons or neutrons spin about an axis and can be aligned along the direction of a magnetic field. This characteristic is vital because it causes nuclei to precess when tipped from alignment with the main magnetic field. The 1H proton has been most widely applied in MRI because of its natural abundance; therefore, this discussion will refer to hydrogen-based MRI. The MRI signal originates primarily from the hydrogen of water and less so from the small hydrogen content of lipids. The actual appearance of the image is affected by a variety of physical parameters. Some of these parameters are characteristic of the tissue sampled and others relate to the MRI sequences. An essential difference between MRI and other modalities is the control that the user has over how data are generated and utilized. The agent of this control is k-space (Fourier space), the platform onto which data are acquired, positioned, and then transformed into the desired image (14). Raw image data are obtained during sampling through k-space, which depicts the spatial frequency domain. High spatial frequencies encode fine details, whereas overall contrast is encoded in the lower frequencies. Data are acquired at discrete intervals to fill k-space as signal amplitude is stored as a function of the spatial frequencies along the read-out and phase-encoding directions. An
image is simply the inverse Fourier transform of the sampled k-space (15).
image is simply the inverse Fourier transform of the sampled k-space (15).
MRI uses high-power static magnetic fields and radiofrequency (RF) pulses to generate tomographic images. Application of weak RF-modulated pulses of a specific frequency will partially align the magnetic moments of protons within the tissue sample against the magnetic field and will induce their resonance; the effect of this RF field is maximal when the nuclei have been deflected by 90°. When the RF pulse ceases, the protons return to equilibrium. During this process, they emit the RF energy of the same frequency, which is then Fourier transformed by a computer into a spatially accurate image whereby differences in signal intensity result in differences in gray levels. The signal intensity depends not only on hydrogen density, but also on the longitudinal relaxation time (T1), reflecting the rate of realignment with the external magnetic field (enhancing the signal), and on the transverse relaxation time (T2), indicating the rate at which nuclei lose coherence with each other (degrading the signal). In normal myocardium, T2 is much shorter (i.e., 60 msec at 1.5 T) than T1 (i.e., 500 msec). The characteristic T1 and T2 relaxation times are exploited to distinguish between normal tissues and to characterize disease processes. In general, MRI contrast improves with increasing hydrogen density, shortening of T1, and lengthening of T2.
Basically, an MRI scanner consists of five major parts: magnet, transmitter, antenna, receiver, and computer. Most modern clinical MRI scanners have magnets consisting of liquid helium–cooled superconducting solenoids operating at a field strength of 0.1 to 3.0 T and with a bore size of approximately 1 m; MRI is best performed with a magnet with higher field strength and homogeneity to improve the signal-to-noise ratio. The transmitter is used for transmitting RF pulses to an antenna or coil, which in turn transmits RF power to the patient and also receives the returning signal. The coil usually surrounds the patient or may be placed directly on the patient’s surface, depending on whether information is required from the whole body or from a selected organ of interest; although surface coils provide higher sensitivity and, therefore, excellent spatial localization of signal, they have the disadvantage of an inhomogeneous RF field, which produces an inhomogeneous signal intensity distribution on images. The receiver amplifies the signal picked up by the coil, and the signal is processed by a computer, which is also needed to operate the entire MRI system.
Specific Magnetic Resonance Imaging Techniques
A detailed discussion of MRI physics is beyond the scope of this chapter, but can be studied from many dedicated textbooks. We briefly discuss some features, including the commonly used imaging sequences in cardiac imaging.
Spin-Echo Sequence
Conventional spin-echo (SE) imaging was for a long time the workhorse of cardiac MRI studies. It has been supplanted by more modern techniques that allow for faster imaging. Nonetheless, spin-echo is still frequently performed with T1 weighting; T2-weighted sequences can be used to demonstrate tumors, inflammation, or myocardial tissue abnormalities. A typical cardiac MRI study is initiated by acquiring scout views through the chest. This is accomplished in a very short time interval, using a fast MRI pulse sequence that has a moderate spatial resolution. The scout views are used to prescribe a volume of interest that is studied with subsequent stacks of SE slices. Each stack has a different orientation (often the three orthogonal planes: coronal, sagittal, and axial) and is acquired in 3 to 5 minutes. Image (slice) thickness, gap, and orientation can be freely chosen. With SE MRI, flowing blood usually generates no signal, whereas myocardium and fatty tissue produce intermediate and high signal intensity. SE MRI is ideal for visualizing morphology, but its limited time resolution does not easily allow functional analysis. The turbo (fast)-spin-echo sequence (TSE) is a faster version of the spin-echo sequence. Instead of one spin-echo pulse, the 90° excitation pulse is followed by a series of 180° pulses that produce several echo signals, each with a different phase encoding. This means that instead of one k-line, several k-lines are measured. The number of 180° pulses is also referred to as the turbo factor. The acquisition time can be shortened with respect to spin-echo imaging by the turbo factor. The most frequent application of TSE sequences is in acquisitions with T2 contrast, in which high turbo factors can be used and the greatest reduction in measuring time can be achieved.
Gradient-Echo Sequence
Gradient-echo (GRE) imaging is a faster technique. In contrast to SE, orderly blood flow generates high signal intensities in GRE imaging. GRE is fast enough to “catch” the signal from previously excited magnetic spins in the blood volume of the image section under study before the blood flow has moved the spins out of the image section. With GRE, the same section can be measured with high repetition rate, enabling the reconstruction of a cine loop of that particular section. This can be achieved in the axial, long axis, short axis, or any desired plane, with a time frame interval of less than 25 msec. GRE can thus be used to detect turbulent blood flow occurring in stenosis, regurgitation, or shunts. Such lesions become particularly obvious when viewing the tomographic section in a cine loop. Turbulent blood flow causes loss of the signal, so stenosis, regurgitation, or shunt flow is detected by a jet of signal void. Cine GRE MRI can be used to assess left (LV) and right (RV) ventricular function in terms of volumes and myocardial mass without geometric assumptions regarding the shape of the ventricles (16). A complete stack that encompasses the heart consists of 10 to 12 adjacent GRE images (typically of 10 mm thickness), each with its own set of time frames, and can be acquired in 10 to 15 minutes. End-diastolic and end-systolic volumes can be measured, or if desired a complete ventricular time–volume curve can be obtained. Another modification of the gradient-echo sequence is the segmented (multishot) gradient-echo sequence, in which only a segment of the image is acquired. In order to achieve all segments, measurements must be made over several RR intervals. Each segment (sometimes also referred to as a “shot”) comprises a certain number of slice-selective RF pulses followed by phase encoding and signal measurement. The segments are distinguished from each other by different values of the phase-encoding gradients (ky values) and consequently provide different k-lines. The newer balanced steady-state free-precession sequences give the best image quality, particularly if the repetition and echo times are kept very short and there has been adequate shimming of the static magnetic field to the region of the heart (17).
Magnetic Resonance Imaging Prepulses
All of the basic pulse sequences (SE, GRE, TSE) can be extended by a prepulse that is transmitted before the actual excitation pulse. The prepulse can consist of one or more RF pulses, sometimes in combination with gradient switching (slice selection, dephasing, etc.). Prepulses can be used for various purposes, such as influencing the contrast and suppressing fat or blood signals. A 180° pulse (inversion pulse) can be used to increase the T1 contrast. The longitudinal magnetization is inverted, and the T1 relaxation does not start at zero, as in the case of a 90° pulse, but at –1. In other words, the contrast range is doubled. The strength of the T1 contrast can be controlled
by the interval between the inversion pulse and the excitation pulse, known as the inversion time (TI). In addition, TI can be chosen in such a way that the magnetization of a tissue during excitation is equal to zero, so that the signal from the corresponding tissue disappears. In this way, the fat signal can be suppressed by using a short TI (e.g., short inversion time inversion recovery [STIR] sequence), or a longer TI can be used to suppress the fluid signal (e.g., fluid-attenuated inversion recovery [FLAIR] sequence). An inversion pulse can be combined with all of the basic pulse sequences. In segmented gradient-echo sequences, the interval between the inversion pulse and the k-line determining the contrast of the shots (ky = 0) is also referred to as the prepulse delay (pp delay). Similarly, a 90° pulse can be used to increase the T1 contrast. In ECG-triggered acquisitions, the relaxation state of the longitudinal magnetization depends on the RR interval. It can therefore have varying values in arrhythmic patients with RR intervals of differing length. If a 90° pulse is transmitted by systole, so that the longitudinal magnetization is reduced to zero, the same T1 relaxation state is ensured after the pp delay, regardless of arrhythmias.
by the interval between the inversion pulse and the excitation pulse, known as the inversion time (TI). In addition, TI can be chosen in such a way that the magnetization of a tissue during excitation is equal to zero, so that the signal from the corresponding tissue disappears. In this way, the fat signal can be suppressed by using a short TI (e.g., short inversion time inversion recovery [STIR] sequence), or a longer TI can be used to suppress the fluid signal (e.g., fluid-attenuated inversion recovery [FLAIR] sequence). An inversion pulse can be combined with all of the basic pulse sequences. In segmented gradient-echo sequences, the interval between the inversion pulse and the k-line determining the contrast of the shots (ky = 0) is also referred to as the prepulse delay (pp delay). Similarly, a 90° pulse can be used to increase the T1 contrast. In ECG-triggered acquisitions, the relaxation state of the longitudinal magnetization depends on the RR interval. It can therefore have varying values in arrhythmic patients with RR intervals of differing length. If a 90° pulse is transmitted by systole, so that the longitudinal magnetization is reduced to zero, the same T1 relaxation state is ensured after the pp delay, regardless of arrhythmias.
Another prepulse that is applied in cardiovascular diagnosis is the black-blood pulse, which is used to suppress the blood signal. The black-blood pulse consists of a series of two 180° pulses. The first pulse is non–slide selective (so-called block pulse). It inverts the magnetism in the total range of the transmission coil (in heart examinations, this can be the whole chest). The second 180° pulse, which is transmitted after the first, is slice selective, and returns the magnetization in the acquisition slice back to its original value. The magnetization of blood flowing into the acquisition slice begins to relax and, after a delay dependent on TR (or the heart frequency), is equal to zero. If the contrast-relevant values of the sequence are measured at this point, the blood signal will be suppressed. The black-blood pulse is often used with TSE sequences (T1 and T2 contrast).
Myocardial Tagging
Regional myocardial function is best assessed using a unique MR technique called myocardial tagging (12), commonly acquired by spatial modulation of magnetization (18). Spatial modulation of magnetization is obtained by applying a radiofrequency prepulse perpendicular to the imaging plane. This prepulse induces local changes in saturation within their planes that label the heart muscle with a dark grid and enables three-dimensional analysis of cardiac rotation, strain (in the subendocardial, mid-wall, and subepicardial layers), displacement, and deformation of different myocardial layers during the cardiac cycle (19). The tags can be applied immediately after the R wave on the electrocardiogram to image systolic function or in late systole to image diastolic function. However, until recently it had not been widely used in clinical cardiac imaging, mainly because of the extensive postprocessing that is required because of the vast amount of information produced by MR tagging series. Recently developed postprocessing software has significantly reduced the time it takes to analyze these tags, making the technique clinically viable (20).
Conventional Magnetic Resonance Angiography and Blood Flow Quantification
MR angiography (MRA) techniques can be divided in time-of-flight (TOF) MRA, phase-contrast angiography (PCA), and contrast-enhanced MRA.
Time-of-Flight Magnetic Resonance Angiography
This is an older technique, which has been used less frequently since the emergence of contrast MRA. In gradient-echo sequences, the longitudinal magnetization of stationary tissue is reduced by repeated excitations. Due to the saturation of the longitudinal magnetization, the signal from the stationary tissue is low. When the blood vessel runs perpendicularly through the slice, “fresh” unsaturated blood (not previously excited, with full longitudinal magnetization) will produce the maximum signal in the excited slice. The overall result is higher contrast between the flowing blood and the saturated stationary tissue. If many overlapping slices are combined, or if 3D techniques are used, inflow MRA can also be used to cover larger volumes.
Phase-Contrast Angiography
With the use of this technique, blood flow velocity is encoded in the phase of the MRI signal and phase changes occur linearly with changes in velocity. An image displaying the phase values across a vessel lumen can therefore be regarded as a velocity map. The signal intensity on quantitative PCA images increases toward bright or dark, depending on the direction of the flow, linearly with the velocity. PCA can be performed across a perpendicular vascular cross section, which allows assessment of instantaneous peak velocity and (spatial) average velocity at frequent time points during a cardiac cycle. Alternatively, to study the jet across a stenotic lesion, the image plane can also be chosen along the longitudinal axis of a vessel. Because true spatial average velocity can be measured, volumetric flow (mL/second) can be calculated by multiplication with the corresponding vascular cross-sectional area. This unique feature of MRI is superior to deriving mean velocity from a sample volume as in Doppler echocardiography. The contour of a vascular cross section is traced by hand, or preferably automatically, using commercially available computer software. The contour is adjusted, if necessary, for changes in position and diameter in every time frame. From these data, velocity and volume of flow can be plotted against time, and stroke volume can be calculated. This technique is being used as an elegant and reliable method for quantifying aortic and pulmonary blood flow because it enables quantification of intracardiac shunts, valvular regurgitation, or differential pulmonary blood flow (21,22
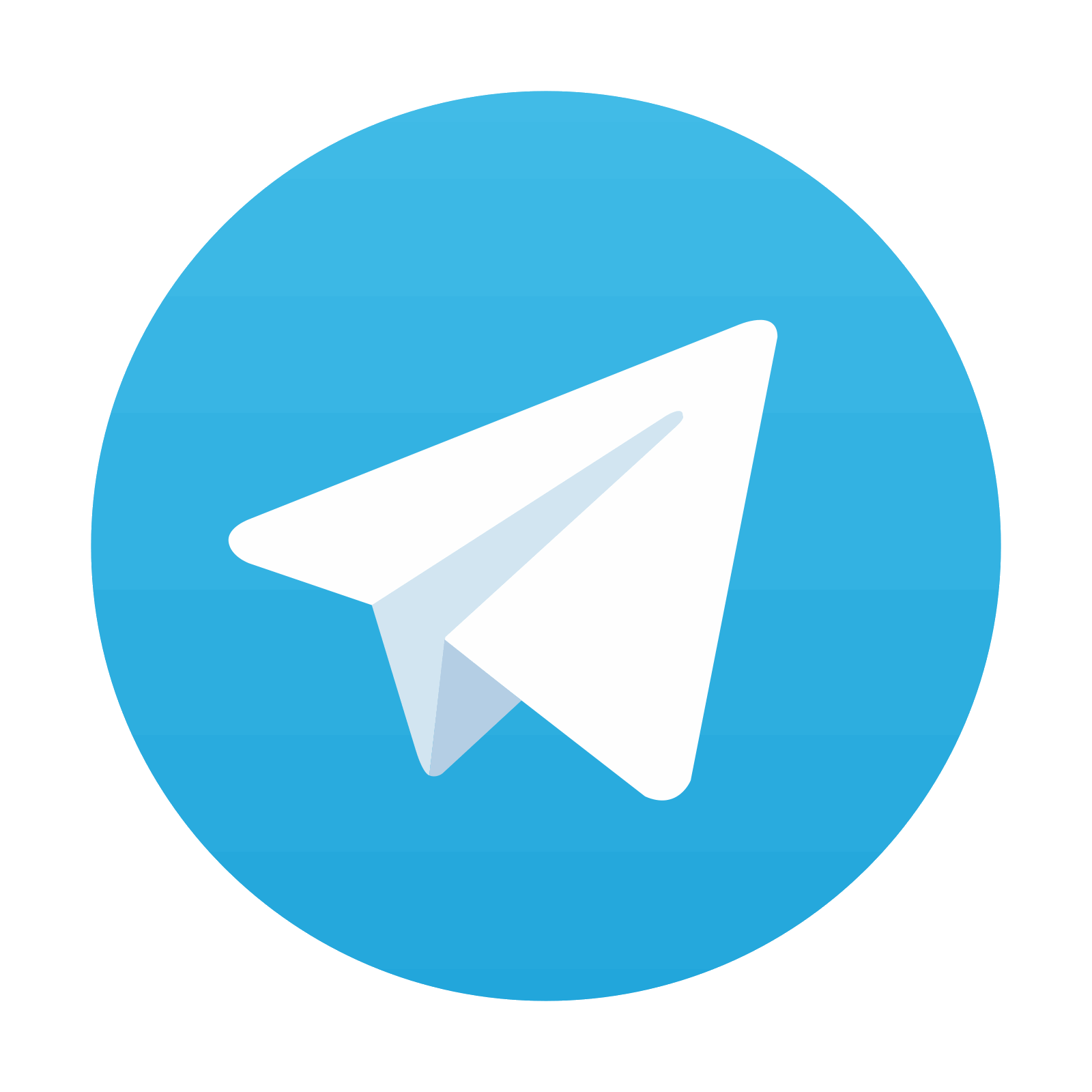
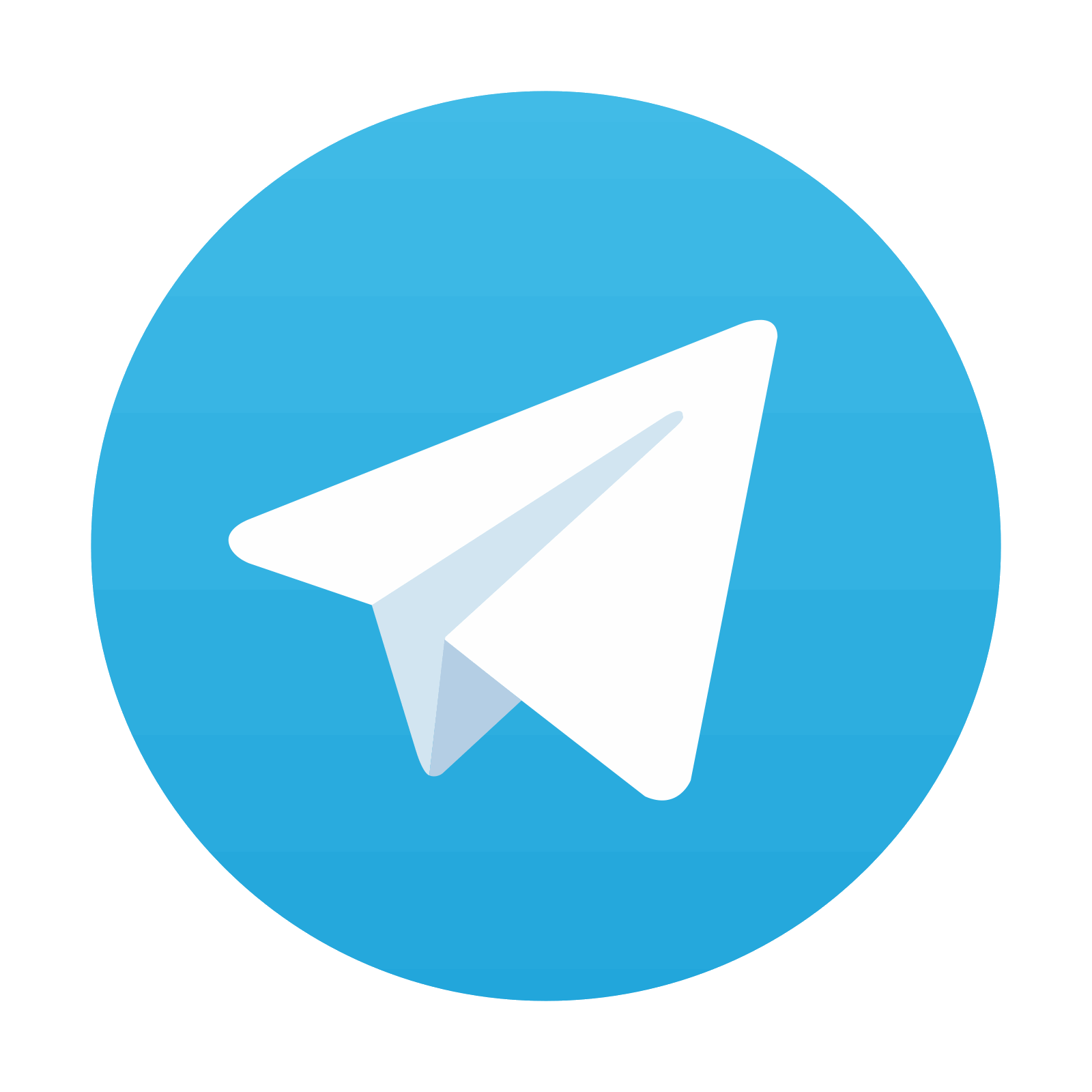
Stay updated, free articles. Join our Telegram channel
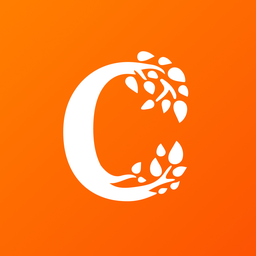
Full access? Get Clinical Tree
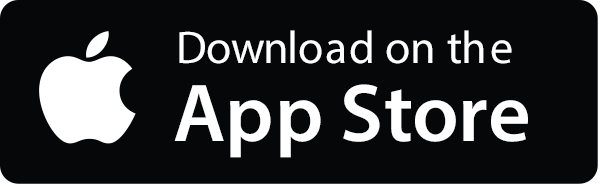
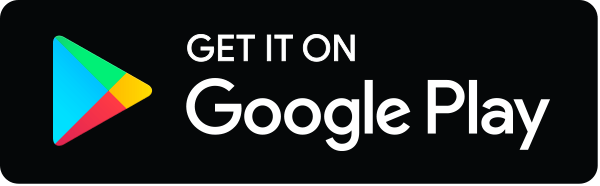
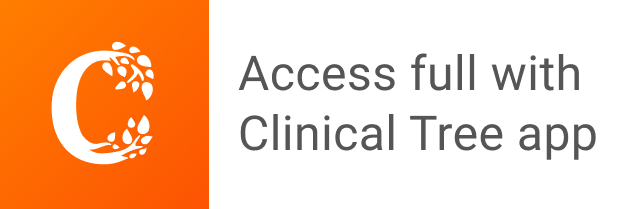